Functional probes for cardiovascular molecular imaging
Introduction
Cardiovascular diseases (CVDs) are considered the most threatening disorder among the global population and are a source of challenge in modern research and medicine (1). There is an urgent need to formulate sensitive and reproducible non-invasive technologies for early disease detection, more specific biomarkers and personalized treatment of CVDs. Many traditional medical imaging methodologies, such as computed tomography (CT), ultrasound, and magnetic resonance imaging (MRI), have been regularly employed to facilitate early diagnoses. These methods serve as a means to supervise early onset of CVDs. Since molecular differentiation is the basis of pathogenesis, traditional cardiovascular imaging technology has aimed at detecting a specific molecular target and fundamental biological development in CVDs (2). Accordingly, in recent years, molecular imaging technologies have been generally accepted in the role of rapid proliferating research interests that significantly promote the non-invasive diagnostic imaging for the conversion of anatomical description to detect specific tissue epitopes and monitor the fundamental biological developments at the cellular and subcellular level (3). Molecular imaging was created as a new branch of biomedical science by merging the areas of imaging technology and probe utilization, thus enabling the direct/indirect spatio-temporal evaluation of molecular markers. In addition to this capability, molecular imaging can provide critical information for advanced diagnosis, appropriate treatment, better prognosis, improved staging, and better management—adding a significantly greater effectiveness to personalized medicine overall (4).
Cardiovascular molecular imaging technologies have been integrated with advanced scientific tools; this merger potentially allows for both morphological and functional imaging in cardiovascular pathophysiology. The emergence of cardiovascular molecular imaging provides detailed molecular and cellular level visualization that allows for early diagnostics and advanced therapeutics practices, which will additionally facilitate a greater understanding of the fundamental biological developments of CVDs. The mutual contribution among inter-disciplinary branches of science, such as molecular biology, chemistry, nanotechnology, and imaging technology, has effectively enhanced the rapid growth in the cardiovascular molecular imaging technologies and has allowed for the development of a large number of attractive new probes in the last decade. The appropriate probes should possess imaging, targeting, and therapeutic functions in order to facilitate the appropriate remedies in the battle against CVDs (2). In this section, we will first briefly describe the general working principles of molecular imaging-probe design, and then summarize the current state-of-the-art in probe-assisted cardiovascular molecular imaging.
Typical molecular imaging probes
Molecular imaging probes are agents that effectively facilitate visualization, quantification and characterization of biological processes in living systems. A molecular imaging probe typically comprises three key components: (I) an imaging agent for the corresponding imaging modality; (II) a targeting moiety that recognizes the intended molecular/cellular target; and (III) a linker connecting the imaging agent with the targeting moiety (Figure 1). The imaging agents used include radionuclides for positron emission tomography (PET) and single-photon emission computed tomography (SPECT), gadolinium (Gd) chelates or superparamagnetic iron oxide nanoparticles (SPIO) for MRI, fluorophores or quantum dots (QDs) for optical imaging, and microbubbles for ultrasound imaging. The targeting moiety, which specifically coordinates with the biomarker of a specific biological process, is also globally termed as a targeting ligand. The linker employed in the design of molecular imaging probes helps in merging the imaging agent with the targeting moiety, and has a significant impact on the pharmacokinetics of the molecular imaging probe. Concerning the latter point, many other distinct features are thus required to optimize the imaging quality; on the other hand, however, some probes do not even have the 3 essential components mentioned (5).
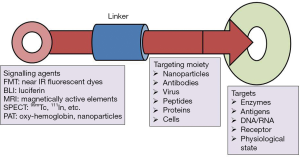
Favorable molecular imaging probes possess useful harness capabilities to gain concomitant anatomic, chemical, and physiological analytical report with high sensitivity, specificity and consistency. To diagnose the biochemical activity of CVDs, particularly at an initial stage, probes need to monitor on the clinical change of a much lower amount of biomarker. Therefore, greater sensitivity is a critical requirement for a potential imaging probe. Amplification strategies such as augmented relaxivity of magnetic substrates or quenching of fluorochromes are the key strategies for developing sufficiently sensitive probes for clinical application. These probes can show a specific physical change that profoundly favors the signal amplification when the probes are spaced close together. In addition, due to the presence of numerous corroding enzymes and proteases in serum/tissue, maintenance of the intact structure of the imaging probes is a prerequisite to achieving high-quality images. The quantitative analysis of the image validity to assure the precession interpretation of physiological and pathological processes of the CVDs, along with the quality of molecular images, can be potentially affected by the in vivo stability of an imaging probe after administration (5).
Initially, the deposition of the imaging probes at the targeted site is required to allow the probes to actively interact with particular biomarkers like enzymes, receptors, and transporters, all which participate in different biological activities correlated with specific molecular/cell compartments. The specific interaction between biomarkers and imaging probes distinctly promotes the acquisition of information from the biological processes at the molecular level, which is effectively used to investigate and understand the distinct biological mechanisms underlying a specific disease. Additionally, the specificity between the imaging probe and the target site can potentially reduce the non-specific site interaction, significantly increasing the feasibility for quantification analysis of imaging output. Antibodies, peptides, aptamers and small organic molecules, have been widely and successfully employed as targeting agents for specific biomolecules in cardiovascular molecular imaging. The use of targeted imaging probes has the capacity to facilitate valuable insights into the pathophysiology of CVDs and to facilitate the development of novel therapeutic strategies (6).
Molecular imaging probes can be considered a special class of pharmaceuticals, especially some theranostics (the fusion of therapeutic and diagnostic approaches). Thus, potentiality and safety are the two most important concerns for molecular imaging probes. Generally, molecular imaging probes are administrated in low dose and their pharmacological manifestation can be negligible. However, the biological effects caused by an imaging probe still require close monitoring. The toxicity of molecular imaging probes is highly dependent on the probes’ physicochemical properties, which include the size, surface charge/coating materials, probe dosage, and duration to probe exposure. In order to enhance the quality of patients’ lives, there is urgent need to synthesize more biocompatible and less potentially harmful imaging probes (7,8).
Strategy for molecular imaging probes design: interdisciplinary efforts
Progress in molecular imaging highly depends on the designing of advanced probes that can effectively detect biological activities on the cellular and molecular level. The design and development of molecular imaging probes require interdisciplinary efforts from the molecular, biological, chemical, imaging and nanotechnology fields (Figure 2). These interdisciplinary efforts can begin with the identification of biomarkers that are applicable to human CVD. Following this, physicochemical expertise is needed to synthesize molecular imaging probes capable of interacting to the target, and to properly optimize the devices for imaging. Multifunctional imaging probes based on nanotechnology can then extend the limits of currently available molecular imaging and allow precision diagnosis, contributing greatly to the advancement of personalized medicine. However, optimism in this new technology must be tempered by consideration of its potentially harmful elements. For instance, many of the fluorophores, contrasting agents and tracers, could be toxic in nature and the right material and specific concentration needs to be first identified before a molecular imaging probe can be deemed safe to administer to patients. More specifically, a gamut of information including cytotoxicity, tissue toxicity, in vivo toxicity and mutagenicity, require documentation in relation to molecular imaging probes before these probes are considered suitable for biomedical application. In this regard, some fluorophores such as indocyanine green and fluorescein have had their toxicity profile’s fully elucidated, and subsequently have been approved by the Food and Drug Administration (9).
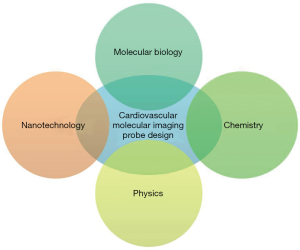
Biomarker-basis of molecular imaging
Biomarkers can act as indicators of health by correlating with disease in physiological measurement, or as determiners of disease complexity, diagnosis and prognosis. In addition, biomarkers also provide signals of fundamental metabolic or pathophysiological activities, or provide pharmacological responses to interventions. The major contributors to biomarker discoveries are molecular profiling studies in cardiovascular medicine, which are based on finding correlations between a molecular signature and a corresponding CVD behavior. Generally, molecular biomarkers include altered/mutant genes, proteins, lipids, and small metabolite molecules, which profiling studies then correlate with a biological behavior or a clinical consequence (10).
The selection of molecular imaging targets should meet certain criteria: They should specifically participate in the disease in question, particularly in CVD, and they should show the difference with respect to the progress and development of the disease. Biomarker determination and selection is therefore the initial, success-limiting step, in the designing of novel molecular imaging probes. As genomic and proteomic information from CVDs becomes increasingly available, databases to find new cellular/molecular biomarkers will provide a plethora of new targets for molecular imaging of CVDs (11). For example, vascular cell adhesion molecule-1, a well-validated marker of endothelial activation of atherosclerosis, could serve as a target for molecular imaging of atherosclerosis (12). Integrins such as avβ3, expressed by neovessels, are similarly employable for molecular imaging of atherosclerosis, and several 18F-labeled affinity ligands have already been developed. The evidence above suggests that the molecular imaging of CVDs based on biomarkers is reaching beyond anatomy to encompass the assessment of aspects of molecular biology related to the pathogenesis of CVDs.
However, CVDs are highly complex and a set of biomolecules are unlikely to be able to detect most of the individual sensitivities related to developing CVD. The development of cardiovascular biomarkers then, naturally needs to overcome various obstacles. Research challenges include the development of probes for molecular imaging that will provide sensitive and robust measurement of thrombosis, infarction, angiogenesis, tissue oxygenation, activation and adhesion of immune cells. The molecular imaging probes also need a scalable synthesis using safe manufacturing processes and the necessary accompanying toxicology studies for human use. Much progress is being made in this field, and the rapidly expanding toolset of available molecular imaging probes will make it possible to non-invasively reveal the biological processes governing CVDs.
Chemistry-molecular probes synthesis
The design and development of a biologically active probe is of utmost importance to realizing the great potential of molecular imaging. By achieving the identification of select molecular biomarkers appropriate for the diagnosis of CVD, advanced chemistry has paved the way in molecular imaging probe synthesis. Specifically, click chemistry has made breakthroughs in a wide array of molecular imaging probe application developments. Within this field, it has become a routine strategy to fine-tune the synthesis of novel molecular imaging probes and to enhance their pharmacokinetic and pharmacodynamics profiles.
Generally, a 1:1 targeting moiety affinity towards the biomarker is considered suitable for molecular imaging. Biochemical amplification strategies are desirable to enhance the imaging signal in the cases where biomarker expression levels are low. The selected targeting moiety should have good affinity, particularly towards the selected biomarker. It also should possess a chemical anatomy that can transport different labels: isotopes for nuclear imaging, Gd for MRI or fluorescence, and dyes for optical imaging. For imaging technologies with intrinsic low sensitivity such as MRI, probes carrying large amounts of Gd allow for high molecular sensitivity (11).
The probe’s in vivo pharmacokinetics, like adsorption, distribution, metabolism, and excretion, are gaining critical attention for their molecular imaging probe design potential. In general, the widely accepted factors that are believed to be significant in the probe’s in vivo pharmacokinetics are its particular physicochemical properties, including ionization constant, lipophilicity and stability. A molecular probe must remain stable enough in the blood circulation in order to effectively access the specifically targeted site with sufficient concentration and time for molecular imaging. Longer systemic circulation does provide greater target exposures that are highly suitable for therapeutic purposes. In cases where the molecular imaging probes are developed to image intracellular tissues, the probe needs to penetrate the cell membrane. Because ionization is a significant parameter that potentially increases/affects the solubility and membrane permeability of a molecule, most of the molecular imaging probes have been developed with ionizable groups and have different charges within the physiological pH range. Moreover, the recent developments in high-performance liquid chromatography/mass spectrometry instrumentation and procedures also provide an array of alternative techniques for determining the metabolites of the imaging probe—a capability which can amply facilitate necessary modification of the imaging probe and potentially enhance its in vivo stability (5,13).
Physics and advanced imaging devices
In CVDs, molecular imaging can diagnose, treat and monitor disease progress. Still, major modifications to these regular procedures need to be adopted in order to upgrade the imaging technologies in light of the concurrent development of more sophisticated molecular probes. At the time of writing, a large number of imaging modalities are under preclinical research, with some of them effectively being ready for investigation at the clinical stage. The predominant imaging modalities can be generally categorized as either anatomical imaging modalities like CT and ultrasound, or functional imaging modalities like PET, single-photon emission computed tomography (SPECT), optical imaging, molecular MRI, and magnetic resonance spectroscopy. From an engineering perspective, each imaging modality would benefit from an increased sensitivity and improved temporal and spatial resolution (2,4).
Anatomical imaging can provide anatomical information that is potentially altered by changes in the anatomy at the time of disease, while functional imaging modalities can determine biochemical activities of CVDs in vivo (2). Each imaging modality can be characterized by its own weaknesses and strengths. By exploiting the combined strengths of different imaging methods, a novel imaging technology might prove to be an attractive alternative to the already mentioned imaging technologies, thereby advancing the capacity to image both small animals and humans. Multimodality imaging was designed by merging different imaging techniques including anatomical, functional and molecular imaging. The sets of information of specific target sites from each imaging technique have been combined to create hybrid images that show characteristics superior to all the previously mentioned imaging technologies. Generally, multimodality imaging modalities are selected to provide synergistic, complementary, or clinically relevant data beyond that furnished by any one of the single-modality methods. For example, co-registration with MRI images provides the anatomic landscape for localizing the functional or molecular information generated by PET (14).
The ideal multimodal imaging approach should provide high precision information like the exact localization, extent, molecular change and metabolic activities of the targeted site, in addition to specifically highlighting the pathogenomic changes which eventually lead to disease. While this is still a comparatively young field, it is reasonable to expect that sometime in the future, the multimodal approach will have significantly advanced cardiovascular molecular imaging technology by formulating novel multifunctional probes. Fresh insights in hybrid imaging technology have already appreciably contributed to translational research in cardiovascular medicine with small and large animals. Nonetheless, the human anatomy’s complex interlinking of organ function, (e.g., when the heart beat is affected by lung motion) yet demands further advancement be made in areas of cardiovascular imaging, such as motion detection devices (15-17).
Nanotechnology is a promising platform for multifunctional molecular imaging probe design
Nanotechnology is a multidisciplinary field and has made great strides forward in recent years. The term “nanotechnology” can generally be applied to any fabrication of new materials with a scale smaller than one hundred nanometers. Unique characteristic features like optical, electronic, magnetic, and chemical reactivity are associated with nanomaterials solely because of their nanoscale sizes, big surface area, and shapes, which can allow for various biomedical applications including molecular imaging. A wide variety of nanoparticles have been used as molecular probes with each imaging modality demanding nanoparticles with specific properties for contrast production. For example, SPIO have long been investigated and are considered to have remarkable potential in MRI contrast enhancement. QDs, which show tunable wavelength photoluminescence from the visible region to near infrared (in accordance with their size), are among the most widely studied nanoparticles for optical imaging (18,19).
Nanoparticle imaging probes offer many advantages compared to conventional small-molecule-based imaging probes. These advantages include producing excellent contrast, lengthy circulation time, and integrating multiple properties. For example, hundreds (or more) imaging labels (fluorescence tags, radionuclides, and other biomolecules) or a group of labels for different imaging modalities, can be merged to formulate a novel single nanoparticle, thus providing drastic signal amplification. In addition, multiple and potentially different, targeting ligands (antibodies, peptides, aptamers, and small molecules) on the nanoparticle show increased binding affinity and specificity (20).
Recently, theranostics have been rapidly developing technology which can be employed as platforms for placing different functionalities not only for molecular imaging functions, but for targeted drug delivery as well. The combination of different imaging labels, targeting ligands, and therapeutics could permit the effective and controlled drug delivery to the targeted site, while providing noninvasive, quantitative monitoring in real time. Application of such multifunctional nanomaterial will potentially elevate the diagnostic validation and design of therapeutic practice, which can then in turn facilitate the prediction of clinical outcomes, realizing the hope of personalized and advance medicine (21). In recent years, the development of theranostic-based nanomedicines has gradually progressed due to the availability of better knowledge about molecular imaging technologies and the processes that occur at the basic molecular and cellular level during cell-exposure to certain therapeutic aids. The intimate understanding of mesenchymal stem cells (MSCs) and related mediated cancer therapy, has facilitated the formulation of theranostic probes which contain umbilical cord-derived MSCs conjugated with triple fusion genes containing the herpes simplex virus truncated thymidine kinase, Renilla luciferase and red fluorescent protein. By using near infrared imaging technology, the real-time activities of the cells can be monitored by bioluminescence signals provided by Renilla luciferase and red fluorescent protein. Hence, the formulated theranostic probe can effectively inhibit breast cancer progression by inducing tumor cell apoptosis and suppressing angiogenesis, in addition to facilitating the tracking of cell delivery and tumor response to MSCs and molecular and cellular activities in tumor cells (22). Similarly, other theranostic probes have been developed by conjugating MSCs with citrate-coated SPIO and investigated for their efficient labeling of human MSCs without transfection agents. The formulated theranostic probes showed no adverse effect in cell proliferation, presentation of typical cell surface marker antigen, and differentiation into the adipogenic and osteogenic lineages. Overall, the synthesized theranostic probes demonstrated very efficient capacity for intracellular magnetic labels for in vivo stem cell tracking by MRI (23).
The most promising applications of nanoplatform-based imaging probes appears to be in cardiovascular medicine. Imaging probes might be able to overcome the barriers of the biological system to accumulate the drug at the targeted site because certain barriers of the biological system potentially affect the drug entry and delivery (24). Still, there remains a significant concern as to the potential adverse effects of human exposure to nanoparticles. The biological distribution and circulation of nanoparticles within biological tissues, and immune responses like phagocytosis, opsonization and endocytosis of nanoparticles, are the specific considerations which seriously need to be to evaluated before the field of nanoparticles can be specialized into nanomedicine proper (19). In the design of probes for molecular imaging, several functionally active nanomaterials that have been employed have very low biocompatibility which can subsequently lead to quick excretion, instability, low circulating time, and potential toxicity. Hence, significant precaution and validation should be taken before adopting the nanomaterial in a biomedical application. PEGylation is widely known as a coating technology that is used effectively for nanoparticle formulation and can potentially enhance the blood circulation time by modulating the protein adsorption to the nanoparticle. The prolonged availability of the nanomaterial in the blood stream significantly augments the accumulation of higher concentrations of prescribed nanomaterial in the targeted site. Moreover, the surface-functionalized nanoparticles with polyethylene glycol (PEG), promote the bio-conjugation with various ligands including drug molecules. Several steps have been taken to reduce the risks posed by nanomaterials in order to enable the novel formulation of highly biocompatible and less toxic nanomedicines (e.g., silica nanoparticles) (25).
Other important questions concerning the application of nanomaterial in molecular imaging still need to be answered before responsible clinical application is possible. These issues include a more thorough understanding of the following: the change that occurs in the association or conjugation between the nano-carrier and the drug molecules, particularly their pharmacokinetics, biodistribution and potential adverse effects of the nanotherapeutic nanomedicine; chance of change of the safety profile of the nanomaterial at the time of conjugation or surface functionalization; the possibility to reduce the toxicity of the polymeric nanoparticles which accumulate considerably at the targeted site and are made up of non-biodegradable polymer and greater than the size of the renal discharge threshold; and the adverse effects (and accompanying prevention measures), that could arise when nanomaterials cross the blood-brain barrier. The above topics are hitherto understudied; thus, clarifying them is critically significant to optimizing the selectivity, efficacy and safety of nanomaterials in clinical practice. Healthy interdisciplinary competition involving the scientific fields of physics, chemistry, biology, nanotechnology, engineering and medicine could contribute to each discipline’s mutual development and the possible design of effective, cutting-edge nanomedicine for the diagnosis and treatment of clinical complications (26,27).
Potential developers of molecular imaging probes need to be aware of the specific properties of functional nanomaterials before they can be repurposed as effective nanoplatforms. The probes should be developed with optimality in features such as surface coating, targeting properties, and extent of biocompatibility, in order to create an assembly with the suitable contrast/therapeutics.
Optical imaging
Optical imaging of live tissues through photonic technology has emerged as a significant tool in advanced biomedical research. Optical imaging can be defined as a technique in which light in ultraviolet, visible or infrared ranges is used to visualize intact organisms. Certain optical imaging techniques like intracoronary optical coherence tomography, Raman spectroscopy and near infrared spectroscopy, are widely employed to inspect vascular cellular and lipid components in patients suffering from CVDs. Fluorescent probes are used to image the molecular phenotype more precisely. Near infrared fluorophores, which have an excitation and emission wavelength of 630 and 1,000 nm, have been employed in optical imaging technologies. Properties like high diffusibility, molecular targeting, and marking for angiogenesis make these fluorophores exceptional candidates for optical imaging technology (28,29). The optical imaging itself has a few advantages over rapid visualization protocols and other simpler methodologies. Fluorescence molecular tomography (FMT) and bioluminescence imaging (BLI) are the two major optical molecular imaging methods that have been employed to diagnose complications of the cardiovascular region. FMT is a recent innovation that permits greater spatial localization of the fluorescence signal, while BLI is another powerful tool for visualizing temporal and spatial development of cardiovascular complications in real time (30).
FMT
In the biomedical research area, microscopic florescence techniques are playing a major role in the study of molecular and cellular development in cell and tissue cultures. Using sophisticated labeling methods, the enhanced spatial resolution provided by florescence techniques, facilitates easy comparison between cellular dimensions and site targeted imaging. Currently, two- and three-dimensional (3D) optical imaging has been employed to investigate the biological activities in intact organisms like mammals. However, this method has come up against certain limitations such as poor light propagation in tissue, and difficulty in reconstruction of exact spatial information due the high scattering and absorbing capacity of biological tissues. As a result, the detection of the signal on the surface of the tissues will be stronger than the signal detected inside the embedded tissues because of the optical properties of the surrounding tissues. In an attempt to overcome these challenges, FMT techniques have been used to map the 3D distribution of a florescent probe or concentration of protein (31). FMT can be defined as any advanced tomographic technique which models the imaging in near infrared regions and facilitates the 3D quantitative detection of a fluorescence signal distribution in the live tissues of the small animal at any depth. Following this, nearly 50,000 to 100,000 pairs of source detectors have been used to measure the diffusion models of photon propagation in turbid media (32). The particularly high sensitivity of these optical imaging techniques potentially permits the detection in tissue of even low concentrations of targeted molecules. Furthermore, these methods are comparatively cost effective, which supports the biomedical research community by reducing their expense in research and development procedures (31). However, the effectiveness of the imaging agents in the in vivo condition could be highly dependent on certain factors such as probe targeting, activation, pharmacokinetics, biocompatibility and photophysics, which are all still not yet fully understood. Advancements in biomedical research have begun to investigate these variable factors as they concern the application of optical reporters for in vivo imaging (33).
A basic explanation of how the FMT method works is as follows. Light passes to the tissues of the animal body at different projections and signals are detected at multiple points in the tissue. The imaging prototype is generated using cylindrical geometry and charge-coupled device (CCD) detection methods. According to the recorded raw emission and excitation information, a synthetic assessment is developed and applied to the inversion code for the reconstruction of absolute fluorochrome density in the animal tissue (Figure 3). With the current available acquisition setup, the determination of threshold fluorochrome is approximately 200 femtomoles in the target tissues volume deep in a phantom enhances the optical properties of the tissues (34). In a separate experiment, a novel handheld device for the 3D fluorescence molecular tomography was described. The device is characterized by its bendable structure and miniaturized size (nearly 10 mm in diameter) which was particularly effective as an intraoperative tool for imaging the internal tissues and facilitating the image guide surgery (35). FMT can overcome the limitations encountered by traditional fluorescent imaging technology, such as poor images at shallow tissues depths due to scattering of signal, by increasing the number of source detectors, resulting in comparatively less background noise and high submillimeter resolution at a depth of 7.5 mm (36).
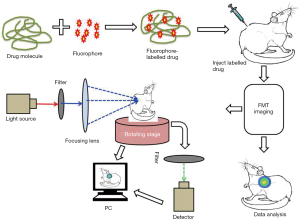
BLI
Novel approaches and technologies that facilitate the early detection of infections, even before the observation of visible clinical manifestations, result in effective diagnosis and treatment, by easing the selection of therapeutic procedures that start at these earlier stages of infection. Fluorescence molecular imaging extends several opportunities to explore the biological processes in intact organisms. Similarly, BLI is a method which works on a sensitive detection of visible light produced during the oxidation reaction between luciferase enzymes and the molecular subtract (30,37). Bioluminescence is the natural capacity some organisms have to convert chemical energy into light energy. Currently, biological researchers are employing this natural phenomenon to visualize intact biological tissues, using a technique called BLI. The basic principle of this technology is the detection of photons emitted from the tissues in the living organisms. In the fluorescence imaging methods, usually light absorption is required to emit light at longer wavelengths; in BLI, light absorption is not required for imaging. For the biological process of bioluminescence to occur, luciferase enzymes are required to be in the presence of luciferin (substrate) and oxygen. In some cases, the enzyme also needs other cofactors like ATP and Mg2+ for successful bioluminescence reactions. Most often bioluminescence emits light at a wavelength of nearly 560 nm, which allows for the imaging of deeper tissue layers with higher resolutions (Figure 4). This method is widely applied in the biomedical areas of monitoring transgene expression, development of infection, toxicology, progression of viral infections and gene therapies (38,39).
The major advantage of the BLI method is its relatively simple implementation which permits the monitoring of a disease with continual quantification of progression of infections, all without scarifying the experimental animal. Moreover, this method decreases the number of experimental animals required in testing because multiple measurements can be recorded within the same animal over the course of infection and treatments, which also minimizes the complication of biological variations. Recent BLI methods have used a variety of luciferase enzymes including firefly luciferase, Renilla luciferase, Gaussia luciferase, Metridia luciferase, Vargula luciferase and bacterial luciferase. Of these luciferase enzymes, firefly, Renilla and bacterial luciferases have been widely employed for optical imaging. The BLI method has gained significantly more importance than its counterpart, fluorescence molecular imaging, mostly due to FMI’s lack of endogenous bioluminescent reaction in mammalian tissues to offer background free images, in addition to the prevalence of fluorescently active compounds in the biological tissues which could affect the target resolution. BLI technology also has different modalities like steady-state bioluminescent imaging, multi-reporter bioluminescent imaging, multi-component bioluminescent imaging and bioluminescence, as a supplementary imaging technique (40). The greater signal-to-noise ration due to the absence of intrinsic bioluminescence background in animal tissues, has promoted BLI as an interesting tool for monitoring biological development of a living animal in real time (41). As such, BLI has been mostly used for small animal studies to monitor and correlate the survival, engraftment and migration of a range of cell populations.
MRI
The basic principle of the MRI works upon the inherent magnetic properties of tissue and the ability to employ these properties to produce tissue contrast. Single protons, which induce the magnetic movement in omni-present hydrogen atoms, produce the magnetic resonance images. As is well-known, when an electric charge in the movement that creates magnetic field, spinning protons on small magnetic field and can be conceived of as little magnet. When a patient is placed in the bore of a large magnet (MRI scanner), hydrogen protons align with the externally applied static magnetic field to generate a net magnetization vector. Most of the protons will distribute randomly on a quantum level, either with or against the scanner static magnetic fields. On the other hand, a little increase in the spins aligns protons with the field causing net tissue magnetization (Figure 5). The time required for this alignment is determined by the longitudinal relaxation time. This longitudinal relaxation time varies between the tissues it is employed to provide contrast for (42). MRI can provide high-spatial resolution anatomic images with intense living tissues’ contrast by using the difference in relaxation times of protons in different biochemical environments. The collaboration of high spatial resolution and contrast, facilitates the anatomic responses of many disease complications to be visualized in animal tissues. MRI can be used to monitor different physiologic parameters like diffusion, permeability and change in blood oxygenation levels after neuronal activation. A recent advancement in nanotechnology is the addition of passive contrasting agents like iron-oxide nanoparticles which can further enhance the contrast (43). MRI molecular imaging data can be evaluated according to the amount of signal change, the area of the tissue displaying contrast enhancement, and the calculation of relaxation time change or direct detection of contrasting agents by multi-nuclear imaging and/or spectroscopy (44).
The MRI method of molecular imaging has certain limitations however, like lower sensitivity than that of nuclear imaging. Nevertheless, certain nanomaterials such as micelles, liposomes, iron oxide nanoparticles and emulsion can be used to deliver contrasting agents like Gd chelates in larger amounts to the targeted sites. In certain cases, iron oxide nanoparticles can be used as contrasting agent in MRI technology (45,46). The application of molecular MRI in vascular imaging is mainly focused on determining the significant components of atherosclerotic plaque, along with adhesion molecules, plaque hemorrhage, ventricular volume or blood velocity, and plaque macrophage content (47,48). MRI molecular imaging comprises a wide range of technologies like site-targeted contrasting agents, drug delivery vehicles, controllable MRI probes, and direct mapping of tissue metabolite (44). However, due to the magnetic field interference caused by devices like defibrillators and pacemakers, MRI cannot be employed on some patients with implantable devices.
SPECT
SPECT is a noninvasive technique that can facilitate 3D functional information with greater resolution, sensitivity and specificity. All nuclear-based imaging technology, including SPECT, relies on the injection of trace amounts of molecules labeled with radioactive isotopes. The decaying of radiolabeled molecules results in the emission of photons. These photons are detected by a position-sensitive detector which can give the arrival location of the photon and its energy. It cannot provide the information about the travelling path of the photons as it requires generating projections of the emitting body. A collimator, a device used to produce a parallel beam of rays or radiation, is inserted between the patient and the detector which in turn shapes the stream of emitting photons into a beam. This allows the detector to locate the photon associated to a line in space along which the decay occurred. Usually, a collimator allows to pass the photons to the detector in a certain angle rest of the photons are removed. This results in the projection of the image at the expense of the discards of most of the photons (49). Customarily, SPECT records the 2D nuclear medicine images obtained at different positions around the patient and gives an estimated 3D radioactivity distribution by employing the tool to reconstruct from multiple projections. SPECT stands out from the other methods of medical imaging modalities due to its special features of instrumentation and image reconstruction (Figure 6) (50).
Generally speaking, energy resolution widely affects the quality of an image. In principle, images should be only constituted with primary photons which are emitted from a decaying radionuclide, and not the secondary scattered photons. A device possessing better energy resolution should able to discriminate the primary photons from the scattered photons and also provide the better image contrast and more precise quantification of radionuclide amount and distribution inside an animal. By using this feature of SPECT in myocardial perfusion imaging, the area of hypoperfusion can be discriminated from its neighboring normal perfusion region by enhancing image contrast (51). SPECT can also be used to detect coronary artery diseases (CAD) even at the time of asymptomatic or moderate CAD. To support this application of SPECT, research has been conducted by the American College of Cardiology Foundation and the American Society of Nuclear Cardiology to assess if SPECT could be used to rate uncertain detection and risk of CAD in asymptomatic patients at initial periods of disease complication. The experimental results demonstrated that, with a retrospectively determined group of asymptomatic patients at moderate CAD risk, SPECT had great potential in detection and risk stratification of CAD. Moreover, the test results showed that average annual mortality was 4% in patients with the high-risk scan but only showed 1.6% in patients with normal scanning. This proves that SPECT imaging can detect the complications in asymptomatic patients and can help in bypass surgery even in the nonoccurrence of symptoms (52). As can be seen, SPECT is a highly sensitive, easy and fast-labeling imaging technology with significant spatial resolution. It can facilitate convenient quantification in vivo and in vitro, provide real-time monitoring of cell viability, and enable imaging over long intervals.
Photoacoustic tomography (PAT)
PAT, also known as optoacoustic imaging, is an emerging imaging technology which holds great promise for preclinical research and clinical treatment. In every imaging technology, the fundamental constraint is the limiting effect of light diffusion on spatial resolution in deep tissue. In contrast to these methods, photoacoustic imaging employs a laser light generated ultrasound, and so has emerged as a promising new technology which can potentially overcome the challenges found in traditional optical imaging. PAT works with the principle of photoacoustic effect where the absorbed optical energy is converted into acoustic energy. Biological tissues are lit by nanosecond laser pulses, raising the temperature at localized places and generating a wideband of ultrasound pulses due to thermal expansion. The light-excited ultrasound pulses spread in the biological tissues and clarify the detection boundary surrounding the biological tissues in ultrasonography (Figure 7) (53). The special properties of acoustic waves, which scatter much less than optical waves in tissues, are what makes PAT technology distinct from other optical imaging technologies. Interestingly, PAT is able to produce high-resolution images in both optically ballistic and diffusive regimes. Moreover, at the time of optical absorption even with single origination, PAT can readily utilize the advantages of rich endogenous and exogenous optical contrasts. For imaging vascular structures, endogenous oxy- and deoxy-hemoglobin are used as anatomical and functional contrasts. Certain dyes, nanoparticles and reporter genes are also used as exogenous contrasts for molecular imaging (33,54-56). PAT imaging has a wide range of applications like glucose metabolism imaging, deep tissue imaging of fluorescent proteins, evaluating the prolong period of biodistribution of an optical contrast agent, and video rate cross sectional imaging (57-60). Due to the advancements in science and technology, PAT has been evolving quickly towards greater spatial resolution, higher frame rates and higher sensitivity detection. Moreover, the continuous progress in PAT has also accelerated and augmented contributions from biology, chemistry and nanotechnology. In summary, PAT has several applications including performing anatomical, functional, molecular and fluid-dynamic imaging at different system levels, and plays a significant role in essential bio-research and clinical practice.
Conclusions
As a new branch of the biomedical sciences, molecular imaging was born under the merging of two areas: imaging and probe technology. Based on the probes allowing direct/indirect spatio-temporal evaluation of molecular markers, molecular imaging can provide critical information for much earlier detection of disease and is expected to have a significant impact in personalized medicine through providing more appropriate treatment, better prognosis, improved staging and better management. This review focused on the development of probes for future applications in novel cardiovascular targeted imaging strategies. The future for probes in cardiovascular molecular imaging rests on, among other innovations, the development of targeted biological molecules, reporter gene methods to enhance gene therapy, tagging cells to track stem cell transplantation, and labeling small-molecule probes with sensitive isotopes. Realizing the potential of these promising probes is likely to play a significant role in both diagnostic and prognostic functions, and the investigation of therapeutic practices.
Acknowledgements
Funding: This work was supported by the National Natural Science Foundation of China (NSFC) (Grant Nos. 81801817, 81422023, 51273165, U1705281, and U1505221), the Major State Basic Research Development Program of China (Grant Nos. 2017YFA0205201, 2014CB744503, and 2013CB733802), and the Program for New Century Excellent Talents in University, China (NCET-13-0502).
Footnote
Conflicts of Interest: The authors have no conflicts of interest to declare.
References
- Langer H, Schönberger T, Bigalke B, Gawaz M. Where is the trace? Molecular imaging of vulnerable atherosclerotic plaques. Semin Thromb Hemost 2007;33:151-8. [Crossref] [PubMed]
- Liu G, Chen X, Ai H. Multifunctional Probes for Multimodality Imaging of Cancer. Molecular Imaging Probes for Cancer Research 2012:863-903.
- Hughes M, Caruthers S, Tran T. Perfluorocarbon Nanoparticles for Molecular Imaging and Targeted Therapeutics. Proceedings of the IEEE 2008;96:397-415. [Crossref]
- Chen K, Conti PS. Target-specific delivery of peptide-based probes for PET imaging. Adv Drug Deliv Rev 2010;62:1005-22. [Crossref] [PubMed]
- Chen K, Chen X. Design and Development of Molecular Imaging Probes. Curr Top Med Chem 2010;10:1227-36. [Crossref] [PubMed]
- Sosnovik DE, Nahrendorf M, Weissleder R. Targeted imaging of myocardial damage. Nat Clin Pract Cardiovasc Med. 2008;5 Suppl 2:S63-70. [Crossref] [PubMed]
- Liu S, Levi J, Cheng Z. General Principles of Molecular Imaging Probe Design. Molecular Imaging Probes for Cancer Research 2012:129-47.
- Liu G, Swierczewska M, Lee S, Chen X. Functional nanoparticles for molecular imaging guided gene delivery. Nano Today 2010;5:524-39. [Crossref] [PubMed]
- Alford R, Simpson HM, Duberman J, Hill GC, Ogawa M, Regino C, Kobayashi H, Choyke PL. Toxicity of Organic Fluorophores Used in Molecular Imaging: Literature Review. Mol Imaging 2009;8:341-54. [Crossref] [PubMed]
- Sajja HK, East MP, Mao H, Wang YA, Nie S, Yang L. Development of Multifunctional Nanoparticles for Targeted Drug Delivery and Noninvasive Imaging of Therapeutic Effect. Curr Drug Discov Technol 2009;6:43-51. [Crossref] [PubMed]
- Schäfers M. The future of molecular imaging in the clinic needs a clear strategy and a multidisciplinary effort. Basic Res Cardiol 2008;103:200-2. [Crossref] [PubMed]
- Preiss DJ, Sattar N. Vascular cell adhesion molecule-1: a viable therapeutic target for atherosclerosis? Int J Clin Pract 2007;61:697-701. [Crossref] [PubMed]
- Alford R, Ogawa M, Choyke PL, Kobayashi H. Molecular probes for the in vivo imaging of cancer. Mol Biosyst 2009;5:1279-91. [Crossref] [PubMed]
- Culver J, Akers W, Achilefu S. Multimodality molecular imaging with combined optical and SPECT/PET modalities. J Nucl Med 2008;49:169-72. [Crossref] [PubMed]
- Cambria E, Pasqualini FS, Wolint P, Günter J, Steiger J, Bopp A, Hoerstrup SP, Emmert MY. Translational cardiac stem cell therapy: advancing from first-generation to next-generation cell types. NPJ Regen Med 2017;2:17. [Crossref] [PubMed]
- Sinusas AJ, Bengel F, Nahrendorf M, Epstein FH, Wu JC, Villanueva FS, Fayad ZA, Gropler RJ. Multimodality cardiovascular molecular imaging, part I. Circ Cardiovasc Imaging 2008;1:244-56. [Crossref] [PubMed]
- Hricak H, Choi BI, Scott AM, Sugimura K, Muellner A, von Schulthess GK, Reiser MF, Graham MM, Dunnick NR, Larson SM. Global trends in hybrid imaging. Radiology 2010;257:498-506. [Crossref] [PubMed]
- Wang X, Liu LH, Ramström O, Yan M. Engineering nanomaterial surfaces for biomedical applications. Exp Biol Med (Maywood) 2009;234:1128-39. [Crossref] [PubMed]
- Liu Y, Miyoshi H, Nakamura M. Nanomedicine for drug delivery and imaging: a promising avenue for cancer therapy and diagnosis using targeted functional nanoparticles. Int J Cancer 2007;120:2527-37. [Crossref] [PubMed]
- Zhang P, Zhang L, Qin Z, Hua S, Guo Z, Chu C, Lin H, Zhang Y, Li W, Zhang X, Chen X, Liu G. Genetically Engineered Liposome-like Nanovesicles as Active Targeted Transport Platform. Adv Mater 2018;30. [Crossref] [PubMed]
- Idée JM, Louguet S, Ballet S, Corot C. Theranostics and contrast-agents for medical imaging: a pharmaceutical company viewpoint. Quant Imaging Med Surg 2013;3:292-7. [PubMed]
- Leng L, Wang Y, He N, Wang D, Zhao Q, Feng G, Su W, Xu Y, Han Z, Kong D, Cheng Z, Xiang R, Li Z. Molecular imaging for assessment of mesenchymal stem cells mediated breast cancer therapy. Biomaterials 2014;35:5162-70. [Crossref] [PubMed]
- Andreas K, Georgieva R, Ladwig M, Mueller S, Notter M, Sittinger M, Ringe J. Highly efficient magnetic stem cell labeling with citrate-coated superparamagnetic iron oxide nanoparticles for MRI tracking. Biomaterials 2012;33:4515-25. [Crossref] [PubMed]
- Lin G, Mi P, Chu C, Zhang J, Liu G. Inorganic Nanocarriers Overcoming Multidrug Resistance for Cancer Theranostics. Adv Sci (Weinh) 2016;3. [Crossref] [PubMed]
- Shamili K, Rajesh EM, Rajendran R, Madhan Shankar SR, Elango M, Abitha Devi N. Colloidal Stability and Monodispersible Magnetic Iron Oxide Nanoparticles in Biotechnology Application. Int J Nanosci 2013;12. [Crossref]
- Shi Y, Pang X, Wang J, Liu G. NanoTRAIL-Oncology: A Strategic Approach in Cancer Research and Therapy. Adv Healthc Mater 2018;7. [Crossref] [PubMed]
- Wang J, Liu G. Imaging Nano-Bio Interactions in the Kidney: Toward a Better Understanding of Nanoparticle Clearance. Angew Chem Int Ed Engl 2018;57:3008-10. [Crossref] [PubMed]
- Suter MJ, Nadkarni SK, Weisz G, Tanaka A, Jaffer FA, Bouma BE, Tearney GJ. Intravascular optical imaging technology for investigating the coronary artery. JACC Cardiovasc Imaging 2011;4:1022-39. [Crossref] [PubMed]
- Lindner JR, Sinusas A. Molecular imaging in cardiovascular disease: Which methods, which diseases? J Nucl Cardiol 2013;20:990-1001. [Crossref] [PubMed]
- Brock M. Application of bioluminescence imaging for in vivo monitoring of fungal infections. Int J Microbiol 2012;2012. [Crossref] [PubMed]
- Stuker F, Ripoll J, Rudin M. Fluorescence molecular tomography: principles and potential for pharmaceutical research. Pharmaceutics 2011;3:229-74. [Crossref] [PubMed]
- Zilberman Y, Kallai I, Gafni Y, Pelled G, Kossodo S, Yared W, Gazit D. Fluorescence molecular tomography enables in vivo visualization and quantification of nonunion fracture repair induced by genetically engineered mesenchymal stem cells. J Orthop Res 2008;26:522-30. [Crossref] [PubMed]
- Gao D, Liu Y, Wang Y, Yuan Z. Protein-modified ultra-small gold clusters for dual-modal in vivo fluorescence/photoacoustic imaging. Quant Imaging Med Surg 2018;8:326-32. [Crossref] [PubMed]
- Ntziachristos V, Tung CH, Bremer C, Weissleder R. Fluorescence molecular tomography resolves protease activity in vivo. Nat Med 2002;8:757-60. [Crossref] [PubMed]
- Yang H, Bin He, Dai X, Satpathy M, Yang L, Jiang H. FMTPen: A Miniaturized Handheld Fluorescence Molecular Tomography Probe for Image-Guided Cancer Surgery. Photonics 2015;2:279-87. [Crossref]
- Ransohoff JD, Wu JC. Imaging stem cell therapy for the treatment of peripheral arterial disease. Curr Vasc Pharmacol 2012;10:361-73. [Crossref] [PubMed]
- Jenkins DE, Oei Y, Hornig YS, Yu SF, Dusich J, Purchio T, Contag PR. Bioluminescent imaging (BLI) to improve and refine traditional murine models of tumor growth and metastasis. Clin Exp Metastasis 2003;20:733-44. [Crossref] [PubMed]
- Sadikot RT, Blackwell TS. Bioluminescence imaging. Proc Am Thorac Soc 2005;2:537-40, 511-2.
- Badr CE, Tannous BA. Bioluminescence imaging: progress and applications. Trends Biotechnol 2011;29:624-33. [Crossref] [PubMed]
- Close DM, Xu T, Sayler GS, Ripp S. In vivo bioluminescent imaging (BLI): noninvasive visualization and interrogation of biological processes in living animals. Sensors (Basel) 2011;11:180-206. [Crossref] [PubMed]
- Xu T, Close D, Handagama W, Marr E, Sayler G, Ripp S. The Expanding Toolbox of In Vivo Bioluminescent Imaging. Front Oncol 2016;6:150. [Crossref] [PubMed]
- Duran C, Sobieszczyk PS, Rybicki FJ. Magnetic Resonance Imaging. Vascular Medicine: A Companion to Braunwald's Heart Disease (Second Edition). 2013:166-83.
- Catana C, Procissi D, Wu Y, Judenhofer MS, Qi J, Pichler BJ, Jacobs RE, Cherry SR. Simultaneous in vivo positron emission tomography and magnetic resonance imaging. Proc Natl Acad Sci U S A 2008;105:3705-10. [Crossref] [PubMed]
- Winter PM, Caruthers SD, Lanza GM, Wickline SA. Quantitative cardiovascular magnetic resonance for molecular imaging. J Cardiovasc Magn Reson 2010;12:62. [Crossref] [PubMed]
- Liu H, Chu C, Liu Y, Pang X, Wu Y, Zhou Z, Zhang P, Zhang W, Liu G, Chen X. Novel Intrapolymerization Doped Manganese-Eumelanin Coordination Nanocomposites with Ultrahigh Relaxivity and Their Application in Tumor Theranostics. Adv Sci (Weinh) 2018;5. [Crossref] [PubMed]
- Lin G, Zhang Y, Zhu C, Chu C, Shi Y, Pang X, Ren E, Wu Y, Mi P, Xia H, Chen X, Liu G. Photo-excitable hybrid nanocomposites for image-guided photo/TRAIL synergistic cancer therapy. Biomaterials 2018;176:60-70. [Crossref] [PubMed]
- Yang C, Tian R, Liu T, Liu G. MRI Reporter Genes for Noninvasive Molecular Imaging. Molecules 2016;21. [Crossref] [PubMed]
- Osborn EA, Jaffer FA. The advancing clinical impact of molecular imaging in CVD. JACC Cardiovasc Imaging 2013;6:1327-41. [Crossref] [PubMed]
- Wang J, Mi P, Lin G, Wáng YX, Liu G, Chen X. Imaging-guided delivery of RNAi for anticancer treatment. Adv Drug Deliv Rev 2016;104:44-60. [Crossref] [PubMed]
- National Research Council. Mathematics and Physics of Emerging Biomedical Imaging. Washington, DC: The National Academies Press, 1996. Available online: https://doi.org/. [Crossref]
- Holly TA, Abbott BG, Al-Mallah M, Calnon DA, Cohen MC, DiFilippo FP, Ficaro EP, Freeman MR, Hendel RC, Jain D, Leonard SM, Nichols KJ, Polk DM, Soman P. American Society of Nuclear Cardiology. Single photon-emission computed tomography. J Nucl Cardiol 2010;17:941-73. [Crossref] [PubMed]
- Khandaker MH, Miller TD, Chareonthaitawee P, Askew JW, Hodge DO, Gibbons RJ. Stress single photon emission computed tomography for detection of coronary artery disease and risk stratification of asymptomatic patients at moderate risk. J Nucl Cardiol 2009;16:516-23. [Crossref] [PubMed]
- Sun Y, Jiang H, O’Neill BE. Photoacoustic Imaging: An Emerging Optical Modality in Diagnostic and Theranostic Medicine. J Biosens Bioelectron 2011;2:108. [Crossref]
- Beard P. Biomedical photoacoustic imaging. Interface Focus 2011;1:602-31. [Crossref] [PubMed]
- Xia J, Yao J, Wang LV. Photoacoustic tomography: principles and advances (invited review). Progress In Electromagnetics Research 2014;147:1-22. [Crossref] [PubMed]
- Xia J, Wang LV. Small-Animal Whole-Body Photoacoustic Tomography: A Review. IEEE Transactions on Biomedical Engineering 2014;61:1380-9. [Crossref] [PubMed]
- Li L, Pang X, Liu G. Near-Infrared Light-Triggered Polymeric Nanomicelles for Cancer Therapy and Imaging. ACS Biomater Sci Eng 2018;4:1928-41. [Crossref]
- Chu C, Lin H, Liu H, Wang X, Wang J, Zhang P, Gao H, Huang C, Zeng Y, Tan Y, Liu G, Chen X. Tumor Microenvironment-Triggered Supramolecular System as an In Situ Nanotheranostic Generator for Cancer Phototherapy. Adv Mater 2017;29. [Crossref] [PubMed]
- Cai W, Gao H, Chu C, Wang X, Wang J, Zhang P, Lin G, Li W, Liu G, Chen X. Engineering Phototheranostic Nanoscale Metal–Organic Frameworks for Multimodal Imaging-Guided Cancer Therapy. ACS Appl Mater Interfaces 2017;9:2040-51. [Crossref] [PubMed]
- Wang X, Zhang L, Wang J, Liu X, Lv P, Zeng J, Liu G. Size-Controlled Biocompatible Silver Nanoplates for Contrast-Enhanced Intravital Photoacoustic Mapping of Tumor Vasculature. J Biomed Nanotechnol 2018;14:1448-57. [Crossref] [PubMed]