Maximum velocity projections within 30 seconds: a combination of real-time three-directional flow MRI and cross-sectional volume coverage
Introduction
Vascular imaging is a well-established element of clinical magnetic resonance imaging (MRI) offering qualitative angiograms for vessel anatomy and quantitative phase-contrast (PC) flow data for vascular function. While pertinent PC studies are usually based on cross-sectional imaging techniques, volumetric flow MRI of a larger view of the vasculature is at the expense of long acquisition times. This holds especially true for 4D flow MRI (1-3) that requires multiple flow-encoding steps, spatial encoding in three dimensions, and the comprehensive sampling of multiple cardiac phases. Although substantial progress has been made to accelerate 4D flow acquisitions to about 2–8 min (4), the measuring time is often followed by a long reconstruction and several post-processing steps including offline visualization and evaluation.
Alternatively, cross-sectional PC MRI—mostly with only unidirectional flow encoding—led to the development of real-time (RT) flow MRI (5-10). An extension to three-directional (3dir) flow MRI was shown feasible (11), but its cross-sectional nature so far hampered the applicability to 3D vascular structures.
This work aims at exploiting a recent development for rapid anatomical volume coverage (VC) (12) to extend RT PC flow MRI to three dimensions. This is accomplished by continuously traversing a 3D volume with cross-sectional RT PC flow MRI and 3dir velocity encoding. The spatiotemporal velocity information of the resulting image series is condensed in maximum velocity projection (MVP) maps that relate to maximum intensity projections (MIP) of flow velocities along the slice dimension. Apart from offering short acquisition times, all reconstruction and processing steps are fully automated and implemented online. First exemplary applications to healthy volunteers deal with flow in the carotid arteries, jugular veins, and intracranial vessels as well as flow along the aortic arch.
Methods
RT flow MRI acquisitions exploit highly undersampled radial and asymmetric gradient-echoes (13) as well as balanced velocity encoding in three perpendicular directions (11,14). For 3D volume coverage successive PC acquisitions are shifted in slice direction (12) by small percentages of the slice thickness to ensure sufficient spatial overlap and temporal sampling of the cardiac cycle. Five different slice propagations were tested corresponding to slice shifts of 1%, 2%, 4%, 8% and 16%, that result in increasingly faster sweeps through the volume of interest. The acquired raw data fills a spatiotemporal hybrid space spanned by cross-sectional k-space datasets at a specific location and time. Detailed acquisition parameters for in vivo studies are summarized in Table 1. Note that for this special acquisition procedure, the volume covered is directly linked to the total image acquisition time. If desired, both can be easily extended by increasing the total number of frames.
Table 1
Parameters | Aorta | Carotids/circle of Willis | Sagittal sinus |
---|---|---|---|
Field-of-view/mm² | 320×320 | 192×192 | 192×192 |
Volume/mm | 40 | 30 | 30 |
Orientation | Sagittal | Sagittal/axial | Sagittal |
Image matrix | 200×200 | 192×192 | 192×192 |
In-plane resolution/mm² | 1.6×1.6 | 1.0×1.0 | 1.0×1.0 |
Slice thickness/mm | 6 | 4 | 6 |
Slice shift | 2% = 0.12 mm | 2% = 0.08 mm | 2% = 0.12 mm |
Venc/cm s−1 | 150 | 80 | 60 |
Repetition time/ms | 3.11 | 4.00 | 3.88 |
Echo time/ms | 2.29 | 3.06 | 3.11 |
Bandwidth/Hz pixel−1 | 1,250 | 1,185 | 1,445 |
Flip angle/° | 10 | 10 | 10 |
Spokes per frame | 4×5 | 4×5 | 4×9 |
Measuring time per frame/ms | 62 | 80 | 140 |
Measuring time per volume/s | 20 | 28 | 30 |
Image reconstructions of vector velocity maps are accomplished as reported in (15). The model-based reconstruction technique poses a non-linear inverse problem (16,17) that is solved by a Gauss-Newton method. The numerical optimization of all four flow-encoding data sets jointly yields (I) a common complex anatomic image, (II) three velocity maps and (III) a set of coil sensitivity maps. Its convergence is improved by numerical balancing of partial derivatives of all unknowns by an automated scaling approach (18). Simultaneously, the build-in frame-wise Maxwell correction (15) dynamically compensates for phase errors by concomitant magnetic fields (19). The serial image reconstruction strongly benefits from temporal regularization, which, for the present case of propagating slices, also emerges as a spatial similarity constraint in slice domain (12). A further refinement of the method refers to the acquisition of initial prep-scans with the body coil to enhance the numerical stability against phase singularities by initializing the inverse reconstruction with a reference phase (20).
In a first post-processing step, color-coded speed maps are calculated for each frame by a root-sum-of-squares operation over all velocity vector components as depicted in Figure 1. In a second post-processing step, a pixel-wise maximum operation is performed along the slice dimension to generate a maximum-intensity projection (MIP) for the T1-weighted anatomic image and seven maximum velocity projection (MVP) maps—one for the speed map and two separate MVP maps for positive and negative velocities of all three directions. These latter maps allow for a discrimination between vessels of different flow direction, e.g., arteries and veins, or the identification of potential backward flow in a single vessel.
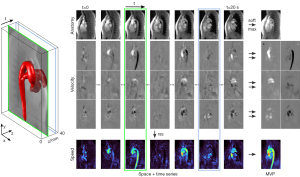
Instead of taking the regular velocity maximum, which returns noisy single pixel data, a smoothed version of the maximum operation is used:
Here, vi are the velocity values ranging from [-π,π], N is the number of frames (or time points) and s is a scaling parameter tuned to s=0.03 π (Figure 2). Similar to related work on softMIP in CT imaging (21) the output of the softmax function is close to the strict maximum for large peak values, but closer to an average for similar or low values such as pure noise. This way, the resulting MVP maps do not rely on single noisy pixels but are subject to an intrinsic denoising and an averaging of peak flow information from multiple heart beats.
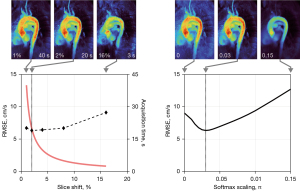
Online reconstruction, post processing and display are ensured by an integrated multi-GPU computer (22) automatically bypassing the reconstruction pipeline of the commercial MRI system, without the need for manual interference. The total reconstruction time per data set is around 1.5 min, resulting in a latency of about 1 min between the end of image acquisition and final display. All results are stored as regular DICOM images in the database of the MRI system.
MRI measurements were performed at 3 T (Magnetom Prisma fit, Siemens Healthineers, Erlangen, Germany). Studies of the carotids and cranial vessels used a standard 64-channel head coil. MRI of the aorta involved an 18-channel thorax coil in combination with suitable elements of a 32-channel spine coil. During technical development, 8 subjects without known illness were recruited among the students of the local university. The study was conducted in accordance with the Declaration of Helsinki (as revised in 2013) and written informed consent was obtained from all subjects prior to MRI according to the recommendations of the local ethics committee. Ethical approval was obtained from the ethics committee of the Goettingen University School of Medicine. The free-running MVP measurements at all listed positions were acquired without cardiac gating or breath holding to maximize patient comfort (free breathing).
As reference for the aorta, one-directional (1dir) RT PC flow MRI (13,17) was performed in axial orientation perpendicular to the aortic 3dir flow MRI study. With the same spatial resolution and a fixed imaging slice, the 1dir results were averaged over 10 heart cycles to minimize noise. The intersection area of the axial 1dir flow and sagittal 3dir flow measurements served as ground truth for the calculation of root-mean-squared errors (RMSE) for velocities in head-feet direction. Comparisons were obtained for different MVP slice shifts and softmax scaling parameters s.
Results
Figure 1 demonstrates the acquisition and post-processing steps for rapid velocity mapping of an exemplary aorta dataset. With use of a slice overlap of 98% (2% slice shift) for consecutive frames, image contrast and quality remain comparable with previous dynamic real-time flow MRI studies of a fixed cross-section (11,15,17). Background noise and residual radial streaking artifacts in the spatiotemporal image series can be ascribed to the pronounced undersampling necessary to achieve sufficient temporal resolution for 3dir velocity encoding. Single frames traversing space and time refer to individual cardiac phases at different positions. This information may be collapsed by a single softmax operation yielding an MVP map that represents the maximum velocity in both space and time. Such maps may be intuitively understood as classic (spatial) MIPs of systolic maximum velocities. The MVP maps project the flow information of a 3D volume onto a 2D map by implicitly exploiting (I) the spatial sparsity of vessels in the body and (II) the temporal sparsity of systolic peak flow.
In comparison to individual frames of the reconstructed spatiotemporal image series (Videos 1,2) the averaging properties of the softmax operation further improve the image quality in terms of reduced streaking artifacts and lower noise levels. Figure 3 illustrates the softmax performance by comparing the MVP map to a single velocity map, the mean over all frames and the regular maximum operation. While the single frame (selected by highest L2-norm) shows incomplete vessel coverage and the mean operation results in velocities that are averaged out, both maximum operations capture the maximum flow information of interest. In the softmax map, the phase noise in static areas is greatly reduced and therefore yields a stronger flow to no-flow contrast. High velocity values are only slightly compromised as revealed by the pixelwise scatter plot. This may be explained by averaging data of non-identical heart beats.
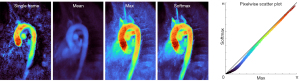
Figure 2 summarizes the MVP results obtained with different slice shifts and softmax scalings. While larger slice shifts greatly reduce the total acquisition time, the RMSE increases due to a growing underestimation of maximum velocities: too fast slice propagations (>4%) run the risk of missing peak systolic flow phases, while too low slice shifts (1%) unnecessarily extend the total acquisition time without adding extra information. Here, the choice of 2% best balances the competing demands of accuracy and short acquisition times.
The softmax scaling allows to tune the degree of averaging of the maximum projection as demonstrated in the right part of Figure 2. While very low values correspond to a strict maximum that depends on single pixels and thus give rise to noise in MVP maps, for too large scaling values the RMSE also increases as maximum velocities are averaged out. Best results are obtained in the intermediate regime at s=0.03 π.
The robustness of the method is characterized in Figure 4 depicting MVP maps of the aortic arch of all 8 subjects. While intersubject differences are apparent, all velocities are in the typical range for young healthy adults. All aorta maps are acquired within 20 s without ECG synchronization or gating strategy and obtained with fully automated processing.
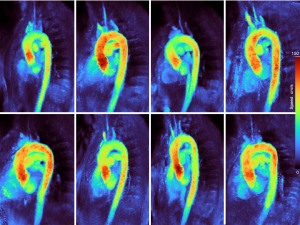
Figure 5 extends the applicability of MVP mapping to vessels in the head and neck regions. These measurements are acquired within 30 s and yield MVP maps with good discernibility of the vessels of interest. In particular, separate MVP maps for positive and negative velocities along the body axis (FH) enable a distinction between arteries and veins.
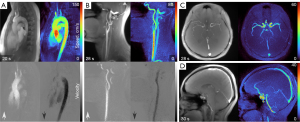
Discussion
This technical report presents a proof-of-concept demonstration of a novel MRI method for rapid mapping of 3dir velocities within a volume. The technique traverses a 3D volume by cross-sectional RT PC flow MRI and condenses the acquired 3dir flow information in MVP maps. While the clinical utility needs to be assessed in future studies, the method may be expected to eventually fulfill the requirements of a quick clinical survey in less cooperative patients. In fact, potential applications may be in pediatric imaging, where rapid anatomical VC has markedly reduced the percentage of infants and young children that had to undergo a sedation for MRI (23). Moreover, short measuring times might open opportunities for repetitive applications to accomplish, for example, dynamic mapping of maximum velocities during physical exercise. From a technical point of view, the approach certainly offers a rapid gating-free localization of the vasculature and promises to facilitate follow-up studies using more detailed flow MRI analyses.
The present work is not without limitations. First of all, the flow information is restricted to maximum velocities and does not provide access to time-resolved hemodynamic parameters as obtained by 4D flow MRI. Instead, the new method can rather be seen in-between full 4D flow MRI and Doppler ultrasonography (US)—combining features and benefits from both worlds: similar to 4D flow, the present method covers a 3D volume and measures velocity vectors in three perpendicular directions, while peak flow velocities are slightly reduced due to the temporal footprint (i.e., the acquisition time) required for 3dir flow encoding. On the other hand, similar to Doppler US, it relies on cross-sectional imaging and provides a simple, fast and robust access to maximum velocities as a well-introduced clinical indicator.
The projection of maximum flow velocities only along the slice dimension is another limiting factor. However, a simple alternative to three-dimensional reformatting is repeating a rapid MVP acquisition along a rotated view. It should also be mentioned that this methodologic pilot study focusses on a qualitative localization of maximum velocities but lacks any reference data and proof of quantitative accuracy. Moreover, while individual frames of the spatiotemporal image series are motion robust, the MVP results can be prone to strong in-plane motion such as deep respiration. Moderate breathing, as performed here, showed only minor detrimental effects, while future measurements might explore breath holding.
At this stage, the lack of general availability must be considered a final limitation, which so far precludes the widespread use and planning of more extended clinical trials. That is because the model-based reconstruction of serial images moving through space with a continuous update of image content and coil sensitivity profiles (15,17,24) poses a nonlinear inverse problem with high computational demand. Consequently, the online applicability requires a GPU computer and dedicated software which is not yet commercially available (25).
Conclusions
The proposed method for rapid mapping of 3dir maximum flow velocities has the potential to serve as a gating-free and quick localization of the vasculature of a volume within 20 to 30 s.
Acknowledgments
Funding: None.
Footnote
Conflicts of Interest: All authors have completed the ICMJE uniform disclosure form (available at https://qims.amegroups.com/article/view/10.21037/qims-22-1126/coif). DV and JF report that they are co-inventors of a patent describing the MRI method used here. The other authors have no conflicts of interest to declare.
Ethical Statement: The authors are accountable for all aspects of the work in ensuring that questions related to the accuracy or integrity of any part of the work are appropriately investigated and resolved. The study was conducted in accordance with the Declaration of Helsinki (as revised in 2013). Ethical approval was obtained from the ethics committee of the Goettingen University School of Medicine and written informed consent was obtained from all subjects prior to MRI.
Open Access Statement: This is an Open Access article distributed in accordance with the Creative Commons Attribution-NonCommercial-NoDerivs 4.0 International License (CC BY-NC-ND 4.0), which permits the non-commercial replication and distribution of the article with the strict proviso that no changes or edits are made and the original work is properly cited (including links to both the formal publication through the relevant DOI and the license). See: https://creativecommons.org/licenses/by-nc-nd/4.0/.
References
- Markl M, Frydrychowicz A, Kozerke S, Hope M, Wieben O. 4D flow MRI. J Magn Reson Imaging 2012;36:1015-36. [Crossref] [PubMed]
- Stankovic Z, Allen BD, Garcia J, Jarvis KB, Markl M. 4D flow imaging with MRI. Cardiovasc Diagn Ther 2014;4:173-92. [PubMed]
- Dyverfeldt P, Bissell M, Barker AJ, Bolger AF, Carlhäll CJ, Ebbers T, Francios CJ, Frydrychowicz A, Geiger J, Giese D, Hope MD, Kilner PJ, Kozerke S, Myerson S, Neubauer S, Wieben O, Markl M. 4D flow cardiovascular magnetic resonance consensus statement. J Cardiovasc Magn Reson 2015;17:72. [Crossref] [PubMed]
- Soulat G, McCarthy P, Markl M. 4D Flow with MRI. Annu Rev Biomed Eng 2020;22:103-26. [Crossref] [PubMed]
- Riederer SJ, Wright RC, Ehman RL, Rossman PJ, Holsinger-Bampton AE, Hangiandreou NJ, Grimm RC. Real-time interactive color flow MR imaging. Radiology 1991;181:33-9. [Crossref] [PubMed]
- Nayak KS, Pauly JM, Kerr AB, Hu BS, Nishimura DG. Real-time color flow MRI. Magn Reson Med 2000;43:251-8. [Crossref] [PubMed]
- Steeden JA, Atkinson D, Taylor AM, Muthurangu V. Assessing vascular response to exercise using a combination of real-time spiral phase contrast MR and noninvasive blood pressure measurements. J Magn Reson Imaging 2010;31:997-1003. [Crossref] [PubMed]
- Joseph AA, Merboldt KD, Voit D, Zhang S, Uecker M, Lotz J, Frahm J. Real-time phase-contrast MRI of cardiovascular blood flow using undersampled radial fast low-angle shot and nonlinear inverse reconstruction. NMR Biomed 2012;25:917-24. [Crossref] [PubMed]
- Sun A, Zhao B, Li Y, He Q, Li R, Yuan C. Real-time phase-contrast flow cardiovascular magnetic resonance with low-rank modeling and parallel imaging. J Cardiovasc Magn Reson 2017;19:19. [Crossref] [PubMed]
- Haji-Valizadeh H, Feng L, Ma LE, Shen D, Block KT, Robinson JD, Markl M, Rigsby CK, Kim D. Highly accelerated, real-time phase-contrast MRI using radial k-space sampling and GROG-GRASP reconstruction: a feasibility study in pediatric patients with congenital heart disease. NMR Biomed 2020;33:e4240. [Crossref] [PubMed]
- Kollmeier JM, Tan Z, Joseph AA, Kalentev O, Voit D, Merboldt KD, Frahm J. Real-time multi-directional flow MRI using model-based reconstructions of undersampled radial FLASH - A feasibility study. NMR Biomed 2019;32:e4184. [Crossref] [PubMed]
- Voit D, Kalentev O, van Zalk M, Joseph AA, Frahm J. Rapid and motion-robust volume coverage using cross-sectional real-time MRI. Magn Reson Med 2020;83:1652-8. [Crossref] [PubMed]
- Untenberger M, Tan Z, Voit D, Joseph AA, Roeloffs V, Merboldt KD, Schätz S, Frahm J. Advances in real-time phase-contrast flow MRI using asymmetric radial gradient echoes. Magn Reson Med 2016;75:1901-8. [Crossref] [PubMed]
- Pelc NJ, Bernstein MA, Shimakawa A, Glover GH. Encoding strategies for three-direction phase-contrast MR imaging of flow. J Magn Reson Imaging 1991;1:405-13. [Crossref] [PubMed]
- Kollmeier JM, Kalentev O, Klosowski J, Voit D, Frahm J. Velocity vector reconstruction for real-time phase-contrast MRI with radial Maxwell correction. Magn Reson Med 2022;87:1863-75. [Crossref] [PubMed]
- Uecker M, Hohage T, Block KT, Frahm J. Image reconstruction by regularized nonlinear inversion--joint estimation of coil sensitivities and image content. Magn Reson Med 2008;60:674-82. [Crossref] [PubMed]
- Tan Z, Roeloffs V, Voit D, Joseph AA, Untenberger M, Merboldt KD, Frahm J. Model-based reconstruction for real-time phase-contrast flow MRI: Improved spatiotemporal accuracy. Magn Reson Med 2017;77:1082-93. [Crossref] [PubMed]
- Tan Z, Hohage T, Kalentev O, Joseph AA, Wang X, Voit D, Merboldt KD, Frahm J. An eigenvalue approach for the automatic scaling of unknowns in model-based reconstructions: Application to real-time phase-contrast flow MRI. NMR Biomed 2017; [Crossref] [PubMed]
- Bernstein MA, Zhou XJ, Polzin JA, King KF, Ganin A, Pelc NJ, Glover GH. Concomitant gradient terms in phase contrast MR: analysis and correction. Magn Reson Med 1998;39:300-8. [Crossref] [PubMed]
- Voit D, Kalentev O, Frahm J. Body coil reference for inverse reconstructions of multi-coil data-the case for real-time MRI. Quant Imaging Med Surg 2019;9:1815-9. [Crossref] [PubMed]
- Meyer H, Juran R, Rogalla P. softMip: a novel projection algorithm for ultra-low-dose computed tomography. J Comput Assist Tomogr 2008;32:480-4. [Crossref] [PubMed]
- Schaetz S, Voit D, Frahm J, Uecker M. Accelerated Computing in Magnetic Resonance Imaging: Real-Time Imaging Using Nonlinear Inverse Reconstruction. Comput Math Methods Med 2017;2017:3527269. [Crossref] [PubMed]
- Sorge I, Hirsch FW, Voit D, Frahm J, Krause M, Roth C, Zimmermann P, Gräfe D. Decreased Need for Anesthesia during Ultra-Fast Cranial MRI in Young Children: One-Year Summary. Rofo 2022;194:192-8. [Crossref] [PubMed]
- Uecker M, Zhang S, Frahm J. Nonlinear inverse reconstruction for real-time MRI of the human heart using undersampled radial FLASH. Magn Reson Med 2010;63:1456-62. [Crossref] [PubMed]
- For availability contact: Jens Frahm, Max Planck Institute for Multidisciplinary Sciences, 37070 Göttingen, Germany. Available online: www.biomednmr.mpg.de