Ratiometric chemical exchange saturation transfer pH mapping using two iodinated agents with nonequivalent amide protons and a single low saturation power
Introduction
pH is an important physiological signal that plays a critical role in maintaining cellular and tissue homeostasis (1). Disturbance of the acid-base balance is a ubiquitous characteristic of many pathophysiological processes (2-7). For example, acidic extracellular pH (pHe=6.5–6.9) prominently features in the tumor microenvironment as a result of deregulated cancer cell metabolism (3,4). The perturbation of kidney pH is also often associated with pathologically changed renal physiology (6,7). Therefore, noninvasive imaging methods for absolute pH measurement have potential utility for disease diagnoses and evaluating responses to pH-dependent therapies (8-12), such as using sodium bicarbonate to alkalinize the tumor microenvironment (13).
Chemical exchange saturation transfer (CEST) magnetic resonance imaging (MRI) has emerged as one of the most promising noninvasive techniques for pH quantification (14-16). Following selective radiofrequency (RF) irradiation, labile protons are saturated, and the saturated protons successively exchange with the surrounding water protons, resulting in a significant loss of the water MRI signal (17-19). The proton exchange process is either base-catalyzed or acid-catalyzed, making CEST MRI suitable for pH imaging. Compared to the sensitivity of MR spectroscopy (MRS), which is another method for detecting protons, CEST MRI is substantially enhanced by two or three orders of magnitude due to repeated saturation and exchange during the pre-saturation period (20-22). To date, a variety of exogenous compounds with exchangeable protons have been developed for pH imaging (15,23-27). To obtain pH maps that are independent of the probe’s concentration, a ratiometric approach has been used in which CEST signals come from two distinct exchangeable protons on the same agent or from two measurements using different B1 saturation powers (14,24,28).
Clinically approved iodinated X-ray agents have been previously studied as diamagnetic CEST (diaCEST) agents for pH imaging (29-35). For example, iopamidol and iopromide possess 2 nonequivalent amide protons resonated at 4.3 parts per million (ppm) and 5.5 ppm from water protons, respectively. During the CEST experiments, these two labile protons are saturated under one power level for ratiometric pH measurement. Tissue pH can be calculated by the ratio of the two CEST signals without the need for concentration of the agent. Longo et al. (29) first used this method with iopamidol to acquire a pH map of the mouse kidney under a B1 of 3µT. Inspired by this study, Chen et al. (32) employed iopromide to measure extracellular pH in a breast tumor model at 2.5 µT. Thereafter, a new power-based ratiometric approach was developed by Longo et al. (24) to assess pH using one amide proton-containing agent, iobitridol, simply by examining the mismatched pH dependencies of the CEST signal at different B1 powers. In that study, two saturation powers of 1.5 and 6 µT were used for renal pH imaging. Recently, Wu et al. (30) further improved the ratiometric method by using the ratios of CEST effects under two power levels (1 and 2 µT) on two labile protons of iopamidol.
Although these studies suggest that ratiometric CEST methods using iodinated agents are advantageous for tissue pH measurement, many factors, including concomitant saturation transfer, acquisition time, sensitivity, and pH detection range, need to be systematically studied and further optimized. First, most ratiometric approaches with iodinated agents have used at least one relatively strong irradiation in their experiments, such as 3 or 6 µT, which can induce serious signal contamination due to water spillover (24). Second, some ratiometric CEST methods incorporate dual saturation power, which doubles the acquisition time and thereby hampers clinical translation. Lastly, the precision of pH measurements for most ratiometric CEST methods in the range above 7.2 to 7.4, the interstitial pH of normal tissues (36,37), still needs to be improved. This may be caused by the fact that amide protons in most iodinated agents exchange too rapidly to be efficiently detected in this pH range.
In this study, five clinically approved iodinated agents and/or their combinations were thoroughly investigated to construct two nonequivalent amide protons for pH imaging. The combination of iodixanol and iobitridol was found to greatly enhance the performance of ratiometric CEST pH imaging under a single low saturation power. The feasibility of in vivo pH mapping was also demonstrated in rat kidneys. We present the following article in accordance with the Animal Research: Reporting in Vivo Experiments (ARRIVE) reporting checklist (available at https://qims.amegroups.com/article/view/10.21037/qims-21-1229/rc).
Methods
Chemicals
Iodixanol [270 mg iodine (I)/mL, Visipaque] and iohexol (350 mg I/mL; Omnipaque) were generously provided by GE Healthcare, (Chicago, IL, USA), with molecular weights (MW) of 1500 Daltons (Da) and 821 Da, respectively. Iobitridol (350 mg I/mL, MW 835 Da, Xenetix; Guerbet, Villepinte, France), iopromide (370 mg I/mL, MW 791 Da, Ultravis; Bayer Healthcare, Berlin, Germany), and iopamidol (370 mg I/mL, MW 777 Da, Isovue; Bracco Imaging, Milan, Italy) were commercially purchased. Low-melt agarose was purchased from Sigma-Aldrich (St. Louis, MO, USA). All other chemicals were of biological grade and were purchased from a local supplier (Aladdin Company, Shanghai, China). Milli-Q water (18.2 MΩ cm−1) was used throughout the experiments.
In vitro phantom studies
A typical phantom containing 6 vials of iodinated agent samples was prepared using a phosphate-buffered saline (PBS). The total amide proton concentration was held constant at 120 mM for all vials, and the pH value was titrated to 5.6, 6.0, 6.4, 6.8, 7.2, and 7.6, respectively, with 5% hydrochloric acid and sodium hydroxide. The pH error in each vial was measured to be less than 0.01 pH/unit with a calibrated pH meter (Mettler Toledo FE28; Mettler-Toledo Instruments, Columbus, OH, USA). The as-prepared sample solutions were placed in 1 mL syringes, inserted into a 50 mL centrifuge tube, and finally sealed with 1% low-melt agarose gel to minimize air.
An initial group of phantoms was prepared with iodixanol and iobitridol, and the mix ratios between 4.3 and 5.5 ppm amide protons in each phantom were adjusted as 2:1, 1:1, and 1:2. Taking 1:1 as an example, the total amide proton concentration of all vials was 120 mM. The corresponding concentrations of iodixanol and iobitridol in each vial were 15 and 60 mM, respectively. The detailed concentrations of 2 agents for each ratio are presented in Table 1. A second group of phantoms with iohexol and iobitridol samples was prepared in the same way as that of the first group. The only difference was the use of iohexol instead of iodixanol to provide 4.3 ppm amide proton. We also prepared two other phantoms with iopromide and iopamidol samples, and the total amide proton concentration and pH range were the same as the previous two groups of phantoms.
Table 1
Mixed ratio (4.3/5.5 ppm) | Iodixanol (mM) | Iobitridol (mM) | Amide proton (mM) |
---|---|---|---|
2:1 | 20 | 40 | 120 |
1:1 | 15 | 60 | 120 |
1:2 | 10 | 80 | 120 |
Before in vitro imaging experiments, each phantom was wrapped with a circulating water heating pad to keep the temperature constant at 37±0.3 ℃ during the imaging period, and the temperature of the phantoms was monitored using an anal temperature detector in real time. All MRI studies were conducted on a 7 T animal MRI scanner (Bruker BioSpin, Ettlingen, Germany). A modified rapid acquisition with relaxation enhancement (RARE) sequence with a short echo time using a continuous wave (CW) pre-saturation pulse was applied for the CEST experiments. The acquisition parameters were as follows: repetition time (TR)/saturation time (TS)/effective echo time (TE) =10,000/5,000/30 ms; field of view (FOV) =30×30 mm2; matrix =90×90; resolution =0.333 mm2; RARE factor =16; slice thickness =5 mm; B1 =1, 1.5, 2, and 2.5 µT. The saturation frequency offsets were set as −10.0, −8.0, −6.0, −5.5, −5.0, −4.3, −4.0, −3.8, −3.6, −3.5, −3.4, −3.6, −3.0, −2.5, −2.0, −1.5, −1.0, −0.6, −0.4, −0.2, 0, 0.2, 0.4, 0.6, 0.8, 1.0, 1.2, 1.4, 1.6, 2.0, 2.2, 2.5, 3.0, 3.4, 3.6, 3.8, 4.0, 4.2, 4.3, 4.4, 4.6, 4.8, 5.0, 5.2, 5.4, 5.5, 5.6, 5.8, 6.0, 8.0, and 10.0 ppm for Mz. We used one offset located at 100 ppm as M0 to normalize the Mz at each offset. To correct the B0 inhomogeneity, a water saturation shift referencing (WASSR) map was collected at a B1 of 0.3 µT and at intervals of 0.02 ppm between ±0.5 ppm.
In vivo MRI studies
All experiments were conducted in accordance with the Guiding Principles in the Care and Use of Animals (China), and were approved by the local Animal Experimentation Ethics Committee of Southern Medical University (No. 2016-0167). Healthy animals were purchased from the specific-pathogen-free (SPF) laboratory at the Animal Experimental Center of Southern Medical University, and were kept in an environment with constant temperature (~25 ℃) and humidity (~50%) with 12-hour light irradiation and 24-hour fresh air provision. Male Sprague-Dawley (SD) rats (n=10; 250±20 g) were randomly divided into two groups using a random order generator in MATLAB (The Mathworks, Inc., Natick, MA, USA), including the iopamidol group (n=5) and the combination of iodixanol and iobitridol group (n=5). The left kidneys of the rats were scanned under anesthesia induced by 3–5% isoflurane, with respiration monitored and gated to suppress motion artifacts. The sample size has been referenced previously in the literature (29,30). The body temperature was maintained at 37±0.3 ℃ using a heating pad and was monitored by an anal temperature detector. A 30 G needle was inserted into the caudal vein for injection of the agent. Scout T2-weighted images were collected with TR/TE=3,000/30 ms, RARE factor =16, and number of excitations (NEX) =2. We also used T2-weighted images to exclude rats with abnormal kidneys; in this study, no rats were excluded. The B0 fields of the left kidneys were carefully shimmed, and WASSR-based B0 maps were used to correct inhomogeneity. Single-slice CEST images along the long axis of the left kidney were scanned during the administration of a mixture of iodixanol and iobitridol (3.374 g I/kg body weight) via a syringe pump. The first half dose of 1.325 mL bolus injection was administered in the beginning, and the rest was continuously infused at 0.11 mL/min during Z-spectra acquisition, to reduce the wash-in/wash-out effect for the pH measurement. The total volume of the mixed agents was 2.65 mL, containing 1.04 mL iodixanol and 1.61 mL iobitridol at concentrations of 0.46 mM and 1.84 mM, respectively. The iopamidol group was injected with the same dosage of exchangeable proton as the combination of iodixanol and iobitridol group. The Z-spectra were acquired under 1.5 µT at unequal intervals and were more numerous at 4.3 and 5.5 ppm (the same as in vitro). TR/TS/TE =6,000/3,000/30 ms; FOV =25×55 mm2; matrix =50×110; resolution =0.50 mm2; slice thickness =1 mm; and RARE factor =32. The total scanning time for the Z-spectra was 10 minutes and 48 seconds. All rats lived normally after the experiments, and no adverse events were observed.
Data analysis
All images were processed in MATLAB using CEST codes downloaded from www.cest-sources.org with some modifications. Before extracting the CEST signals, raw data were interpolated using the spline interpolation with 0.01 ppm intervals smoothly connected to the Z-spectra. Frequency deviation was estimated using WASSR to correct the B0 inhomogeneity. The Z-spectra (Mz/M0) within the ±10 ppm range were normalized by the unsaturated M0 and inverted by the equation:
where Mz and M0 represent the signal acquired with and without saturation, respectively. The Z-spectra were then fitted using the sum of seven Lorentzian functions corresponding to the magnetization transfer (MT) with a super-Lorentzian line shape, direct water saturation (DS) effect, nuclear Overhauser effect (NOE), 2 hydroxyl protons, and 2 nonequivalent amide protons, whose resonance frequencies were located at -1, 0, -3.5, 0.8, 1.8, 4.3, and 5.5 ppm, respectively, as shown in the following equations (38-40):
where Li is the Lorentzian spectrum of the i th pool, and w is the saturation frequency. In the equation, A, w0, and lw are the amplitude, center frequency, and linewidth of the Lorentzian spectrum of each pool, respectively. Specially, the n is the number of fitting pools, with n=4 and n=5 for in vitro Lorentzian fitting with 1 and 2 types of exchange protons after excluding the MT and NOE, and n=7 for in vivo fitting. Multi-pool Lorentzian line shapes were resolved by iterative fittings to minimize the residual error. The saturation transfer (ST) effect values were determined using the fitted amplitudes of each Lorentzian component. A ratiometric measurement was obtained using the ratio of two CEST signals located at 4.3 ppm and 5.5 ppm (41).
For comparison, the ST values were also measured with a magnetization transfer ratio (MTR) asymmetric analysis (MTRasym) using the following equation.
Finally, the log10 ratio of CEST effects from the two nonequivalent amide protons was calculated and linearly fitted with respect to pH. The correlation coefficient (R2) and the sum of squares due to error (SSE) were acquired, and the linearity (k) was regarded as a pH response. The acquired calibration curve was used for both in vitro and in vivo pH measurement. After B0 correction and Lorentzian fitting of the Z-spectra of the kidneys voxel by voxel, the CEST signal maps of the kidneys at 4.3 and 5.5 ppm were separated. Then, the log10 ratio map was calculated using equation (4) and inputted to the calibration curve to obtain the pH maps of the kidneys. Regions of interest (ROIs) were drawn manually from anatomical T2-weighted (T2W) images to measure the pH values of the cortex, medulla, and calyx. Finally, the pH maps were overlaid on T2W images. The quantified pH values were expressed as the mean ± standard deviation (STD).
Statistical analysis
The pH values measured in the cortex, medulla, and calyx were statistically analyzed using a unilateral Student’s t-test (GraphPad Prism 8; GraphPad Software, San Diego, CA, USA). Kolmogrov-Smirnov (K-S) testing was used to affirm the data matched the normal distribution in advance. If the assumptions were not met, non-parametric tests would be processed. A P-value of less than 0.05 (unpaired t-test) was considered statistically significant.
Results
Iodinated agents and CEST properties
Figure 1 shows the chemical structure of five clinically used X-ray iodinated agents investigated in this study. All agents have similar structures, except for iodixanol, which is a dimer. They are all nonionic compounds that are not charged. Specifically, iopamidol and iopromide molecules have two types of nonequivalent amide protons, one resonating at 4.3 ppm and the other at 5.5 ppm downfield from the water proton. There is only one type of amide group in iobitridol, iohexol, and iodixanol, and the corresponding chemical shifts are presented in Figure 1. The number of exchangeable amide protons in each iodinated compound is 1 for iobitridol, 2 for iopromide and iohexol, 3 for iopamidol, and 4 for iodixanol. Thus, to compare their performances with those from previous studies, we quantified their concentrations according to the exchangeable amide proton.

As the CEST properties of iopamidol and iopromide are widely available in the literature (23,24), we only characterized the other three iodinated agents. Their CEST spectra were obtained at different pH values (120 mM in a PBS solution). Iodixanol (Figure S1) and iohexol (Figure S2) had significant CEST effects at 4.3 ppm, whereas the CEST signal of iobitridol (Figure S3) was at 5.5 ppm. The appearance of all CEST signals became broader with an increase in pH, and that of iobitridol changed the fastest among them (Figure S4). After careful examination, we found that the CEST peak of iodixanol was slightly narrower than that of iohexol, especially in the high pH range. These results indicated that the exchange rate of iodixanol amide proton was the slowest, which is consistent with the kex quantification by Longo et al. (34).
CEST spectra and analysis methods
Previous studies have shown that labile protons with a slow kex are more favorable for high labeling efficiency and producing CEST effects under a low saturation power (17). In this regard, iodixanol may be a desirable candidate for pH imaging under a low B1. To construct two nonequivalent amide groups, such as iopamidol, we simply combined iodixanol with iobitridol as our ratiometric pH probe. A phantom containing 6 vials of mixed iodinated agents in the pH range between 5.6 and 7.6 was prepared for pH calibration. Figure 2 displays representative Z-spectra in the pH range of 5.6 to 7.6 obtained under a B1 of 1.5 µT. It was observed that the CEST signals from two different amide protons at 4.3 and 5.5 ppm was resolved at a lower pH (Figure 2C), but the signal at 5.5 ppm was lower at a higher pH (Figure 2E,2F). Lorentzian fitting with five pools, including two amide protons at 4.3 ppm and 5.5 ppm, two hydroxyl protons at 0.8 ppm and 1.8 ppm, and DS, were conducted on the Z-spectra. The entire Z-spectra demonstrated a very good fitting (R2=0.999).
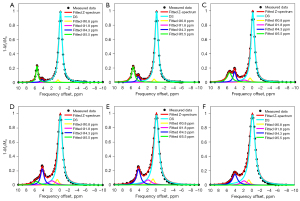
In addition to the Lorentzian fitting analysis, CEST signals from two nonequivalent amide protons were extracted using MTRasym analysis. A log10 ratio based on two CEST signals at 4.3 and 5.5 ppm (hereinafter referred to as the “CEST ratio”) was calculated and linearly correlated with the measured pH. The obtained ratiometric images and correlation results are exhibited in Figure 3. For the Lorentzian method, the pH calibration had an R2 of 0.991 and SSE of 0.057 pH units (Figure 3D). In comparison, the calibration using the MTRasym analytic method had an R2 value of 0.898 and an SSE of 0.118 pH units (Figure 3B). This result suggested that the pH quantification of the ratiometric method using Lorentzian fitting was more appropriate than that of the MTRasym analysis. Lastly, we also compared the performance of the two analytic methods using iopamidol, and the ratiometric images and correlation results are illustrated in Figure 3E-3H. Similar results with iopamidol further supported our previous conclusion that the Lorentzian fitting analysis can provide a more reliable and larger pH-detection range than the asymmetric analysis.
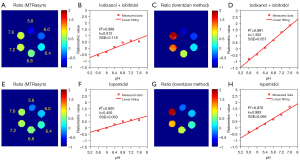
Two nonequivalent amide protons in iodinated agents
We further prepared another phantom using iobitridol and iohexol to compare the performance with that of iobitridol and iodixanol. The mix ratio and the total amide proton concentration were maintained the same. The only difference was that, as the provider for 4.3 ppm amide proton, iohexol was a monomer whereas iodixanol was a dimer. The exchange rate of the iohexol amide proton was relatively fast compared to that of iodixanol. The impact induced by this exchange difference was carefully studied and compared, as displayed in Figure 4. For the combination of iobitridol and iohexol (Figure 4B), the correlation coefficient (R2) and pH response (k) were measured as 0.933 and 1.176, respectively. The precision and sensitivity were inferior to those of the iodixanol and iobitridol combination (Figure 3D). In addition, the pH detection range using iohexol and iobitridol was narrowed to 5.6 to 7.2.
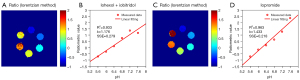
Two nonequivalent amide protons can be provided by 1 or 2 iodinated molecules. To investigate this subtle alteration, we prepared another phantom using iopromide and compared it with the above mixing phantom. As shown in Figure 4D, an R2 value of 0.963 was obtained for the pH calibration curve using iopromide under a B1 of 1.5 µT, and the k value was 1.433. Both the pH response and precision were quite close to that of the combination of iodixanol and iobitridol, even a little better for iopromide in the pH response.
Mixed ratio and B1 saturation power optimization
Based on the methods for CEST signal analysis, we optimized two critical experimental parameters. First, the mix ratios between 4.3 and 5.5 ppm amide protons with iodixanol and iobitridol were tuned to the ranges of 2:1, 1:1, and 1:2. The effect of the mix ratios on the performance of the ratiometric pH imaging is depicted in Figure 5A. For these 3 mixing phantoms, the CEST ratios were found to be linearly correlated with the titrated pH. The R2, SSE, and pH response (k) are listed in Table 2. With the increase in iobitridol amide protons, the correlation coefficient or quantification precision improved and the 1:1 ratio seemed to achieve the best performance; the k deteriorated from 1.461 to 1.253 per pH unit. In contrast, iodixanol amide protons seemed to act in the opposite direction. When the mix ratio reached 2:1, R2 decreased to 0.927. To trade off the precision and sensitivity, a moderate mix ratio of 1:1 was selected to undertake pH imaging of the kidneys in vivo.
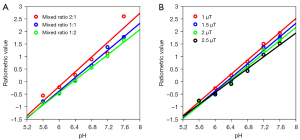
Table 2
4.3:5.5 ppm | R2 | SSE | k | |
---|---|---|---|---|
Mixed ratio | 2:1 | 0.927 | 0.468 | 1.461 |
1:1 | 0.991 | 0.057 | 1.352 | |
1:2 | 0.996 | 0.019 | 1.253 | |
B1 | 1.0 μT | 0.996 | 0.019 | 1.386 |
1.5 μT | 0.991 | 0.057 | 1.352 | |
2.0 μT | 0.984 | 0.067 | 1.302 | |
2.5 μT | 0.981 | 0.076 | 1.187 |
R2, correlation coefficient; k, linearity; SSE, error sum of squares.
We were also interested in determining the minimal RF saturation power that could provide an acceptable calibration curve of CEST ratio versus pH. We tested four irradiation powers from 1 µT to 2.5 µT and the results are presented in Figure 5B and Table 2. It appears that the change in saturation power in the studied range did not have a significant influence on the pH response and precision. With the increase in the level of power from 1 µT to 2.5 µT, the pH response changed from 1.383 to 1.187, and the 1 µT power achieved the best performance, with an SSE of 0.019. Considering that the kex of the iobitridol amide proton was extremely high at pH values above 7.0, the optimal B1 that we chose here was 1.5 µT. It should be mentioned that 1.5 µT was also used by Longo et al. in the power-based ratiometric method with iobitridol (24).
The optimized ratiometric CEST protocol with the iodixanol and iobitridol combination was used to acquire an in vitro pH map based on the CEST ratio and pH calibration curve, and the results are exhibited in Figure 6A. Vials of pH 6.0, 6.4, 6.8, and 7.2 were quantified as 5.97±0.02, 6.38±0.01, 6.78±0.01, and 7.21±0.02, respectively. The pH determined from the ratiometric CEST MRI strongly correlated with the titrated pH by a root mean square error (RMSE) of only 0.021 in the pH range of 5.6 to 7.6 (Figure 6B).
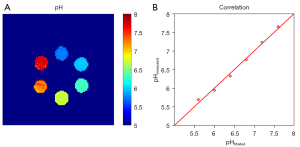
In vivo CEST experiments
We then applied the established protocol for in vitro experiments to measure the pH values in healthy rat kidneys. As iodixanol and iobitridol are freely and quickly excreted by glomerular filtration, there may be some alteration in the mix ratio of nonequivalent amide protons in the kidney after intravenous injection. Therefore, we first used micro-computed tomography (CT) to separately measure the dynamics of iodixanol and iobitridol in healthy rat kidneys (Methods in Appendix 1 and Figure S5). Their concentration in each kidney was then converted into the ratio of nonequivalent amide protons, and finally used to determine a suitable time window for CEST acquisition. As shown in Figure S5, the ratio in the region of the whole kidney was stable with a value of approximately 1 during the period of 6–18 minutes post-injection in the healthy rats (n=8), and the mean value was measured as 0.93±0.13. There was no significant difference between the ratio of different time interval.
The optimal time window was subsequently used for in vivo Z-spectra acquisition. Through intravenous injection of pre-mixed agents at a dosage of 3.375 g I/kg body weight, the Z-spectrum of one rat kidney was obtained under a B1 of 1.5 µT. Typical inverted Z-spectra of the cortex, medulla, and calyx of 1 kidney are shown in Figure 7. Small peaks around 4.3 and 5.5 ppm can be observed clearly in both Z-spectra of the calyx and medulla. The Lorentzian line shape fitting analysis was used to extract two CEST signals, including the NOE pool, in addition to the other 4 pools used in vitro. All layers of the kidney showed a good fit. The resolved CEST signals at 4.3 and 5.5 ppm are depicted in Figure 8A,8B. We can observe that the amplitudes of these two CEST signals increased from the cortex to the calyx. The calculated ratiometric image and the corresponding pH map generated from the calibration curve in vitro are presented in Figure 8C,8D, respectively. The pH values of the cortex, medulla, and calyx were measured as 7.23±0.09, 6.55±0.15, and 6.29±0.23, respectively, which are significantly different from each other. In Figure 8E-8H, iopamidol was also used for pH imaging of the kidney using the same method, and the pH values of the cortex, medulla, and calyx were measured as 7.02±0.06, 6.71±0.10, and 6.20±0.24, for comparison. The pH values of the kidney as measured by the combination of iodixanol and iobitridol were higher than iopamidol and closer to previous study used pH probe (11).
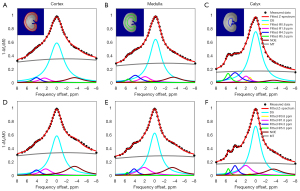
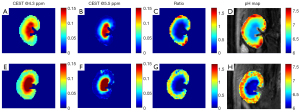
Discussion
Ratiometric CEST pH imaging methods using clinically approved iodinated agents are highly promising, owing to their enhanced sensitivity and their capability to create pH maps that do not need to measure the agent’s concentration in a separate experiment. Their translation potential in human volunteers has also been successfully demonstrated on a 3 T MRI scanner (42,43). In this study, we thoroughly studied the optimal combination of two different nonequivalent amide protons from five clinically approved agents for ratiometric pH mapping. Although the two nonequivalent amide protons in iodinated agents possess a large chemical shift, their frequency of separation is relatively small. This often causes two CEST signals to coalesce when the chemical exchange is rapid at high pH values. Through an MTRasym analysis, as previously used, to partially suppress MT and DS contributions, it is not easy to extract the exact contribution from these two amide protons. The Lorentzian fitting analysis can help distinguish these two signals to some extent, thereby improving quantification in the pH range between 5.6 and 7.6.
The key factor to obtain accurate pH measurements is to tackle the overlapping problem of two CEST effects in the physiological pH range. Compared with using two nonequivalent amide protons obtained from one iodinated agent, combining two agents (such as iobitridol and iodixanol) can benefit from the acquisition of two independent CEST signals at 4.3 and 5.5 ppm from two separated phantoms, such as the experiment depicted in Figures S1-S3. Such a prior signal characteristic can be helpful for the Lorentzian fitting analysis and for extracting the exact contribution when they are mixed together. The precision difference between Lorentzian fitting and MTR asymmetry analysis may be attributed to the fact that the Lorentzian-fitted CEST signal has much more offset information and is thus more stable than that of the asymmetric method with only one offset frequency, which concurs with the findings by Wu et al. (30). This strategy may be more effective on a clinical 3T MRI scanner when the coalescence problem is more pronounced.
Moreover, the performance of ratiometric pH mapping also depends on the extent of matching of the exchange rates of the two nonequivalent amide protons. For example, a previous study adopted a dual B1 of 2 and 1 µT instead of a single low saturation power for ratioing two CEST effects of nonequivalent amide protons in iopamidol, partially owing to the factor of mismatched exchange rates in the physiological pH range. As it is flexible to combine two agents for constructing nonequivalent amide protons, iodinated agents can be chosen with desirable CEST properties. The exchange rate of amide protons in iodixanol, which is a dimer iodinated agent, is slower than that of a monomer (33). As expected, iodixanol was found to be a better choice than iohexol for constructing nonequivalent amide protons with iobitridol. A relatively high pH response (k=1.352), improved precision (R2=0.991), and broader pH detection range (5.6–7.6) were achieved with iodixanol and iobitridol over the iohexol and iobitridol combination (k=1.176, R2=0.933, and pH range 5.6 to 7.2).
Although the in vitro pH quantification results are very promising, there are also some challenges of the proposed method for in vivo pH imaging. The dosage is the first important issue to be addressed. In this study, the injection dosage per amide proton of the mixture of iodixanol and iobitridol was equal to that of iopamidol in a control experiment. However, the dosage per iodine, a general measure of dose when used in X-ray/CT studies, was 2.25 and 1.50 times higher than iopamidol and iopromide, respectively. This is mainly because the number of amide protons in iobitridol is small. For kidney pH imaging, it may not be a serious problem, since most injected agents are cleared from the body by glomerular filtration. However, the dosage would be a significant issue for those organs with limited blood supply.
The other significant issue that should be considered is the pharmacokinetics of the two injected agents. For in vivo applications, the strategy of combining two iodinated agents for ratiometric CEST pH imaging needs to maintain the ratio of nonequivalent amide protons nearly constant during the CEST acquisition period. However, the delivery rates of monomer and dimer iodinated agents to the kidneys can be slightly different and can influence the pH quantification in scenarios where the pharmacokinetics of the two agents are very different. In our study on healthy rats, the ratio of nonequivalent amide protons was 0.93±0.13 in the kidney during a specific time-window of 6–18 min. For a short acquisition period (6–9 min in the CT scheme) of the Z-spectra from 4.3 to 5.5 ppm, the ratio variation was even smaller (1.02±0.08). This slight change in the ratio of nonequivalent amide protons did not significantly affect the pH measurement. The measured pH values for the cortex, medulla, and calyx of the iodixanol and iobitridol combination were 7.23±0.09, 6.55±0.15, and 6.29±0.23 respectively, which was a little higher than for those using iopamidol (Figure 8H: 7.02±0.06, 6.71±0.10, and 6.20±0.24). The gradually decreasing pH values from the cortex to the medulla to the calyx were similar to those reported in the literature (29,30), and in agreement with previously reported values which were measured using gadolinium-based contrast agents (7.3±0.10, 7.0±0.30, and 6.3±0.50 for the cortex, medulla, and calyx, respectively) (11). The mean pH of the entire kidney was 6.84±0.33, which was also comparable with previously reported values (29,30).
Conclusions
The combination of iodixanol and iobitridol at a ratio of 1:1 was found to be suitable for pH mapping. Improved precision and an extended pH detection range were achieved in vitro under a B1 of 1.5 µT. Our results show that the ratiometric CEST method using two iodinated agents with nonequivalent amide protons could be used for in vivo pH mapping of the kidney under a single low B1 saturation power. The strategy used here to tackle the overlapping issues may be helpful for future clinical studies of pH mapping at low fields.
Acknowledgments
The preliminary work was presented as a digital poster at the 29th International Society for Magnetic Resonance in Medicine (ISMRM) Annual Meeting (2021, Virtual Conference, abstract No. 2119). The authors would like to thank Mr. Hongwei Lin from GE Healthcare, Prof. Jun Shen from Sun Yat-sen Memorial Hospital of Sun Yat-sen University, Prof. Xiaodong Zhang from the Third Affiliated Hospital of Southern Medical University, Dr. Chenggong Yan from Nanfang Hospital of Southern Medical University, Dr. Yi Yang from The First Affiliated Hospital of Shenzhen University, and Dr. Hao Wu from Daping Hospital of Third Military Medical University for their kind help in sourcing the iodinated agents used in this study.
Funding: This study was supported in part by the National Natural Science Foundation of China ((Nos. 81871349, 21673281, 31870982), the Technology R&D Program of Guangdong (No. 2017B090912006), the Medical Scientific Research Foundation of Guangdong Province (No. A2018268), the Guangzhou Science and Technology Program (No. 202102080058), and the open project of the CAS Key Laboratory of Nano-Bio Interface (No. 18NBI02).
Footnote
Reporting Checklist: The authors have completed the ARRIVE reporting checklist. Available at https://qims.amegroups.com/article/view/10.21037/qims-21-1229/rc
Conflicts of Interest: All authors have completed the ICMJE uniform disclosure form (available at https://qims.amegroups.com/article/view/10.21037/qims-21-1229/coif). The authors have no conflicts of interest to declare.
Ethical Statement: The authors are accountable for all aspects of the work in ensuring that questions related to the accuracy or integrity of any part of the work are appropriately investigated and resolved. All experiments were conducted in accordance with the Guiding Principles in the Care and Use of Animals (China), and were approved by the local Animal Experimentation Ethics Committee of Southern Medical University (No. 2016-0167).
Open Access Statement: This is an Open Access article distributed in accordance with the Creative Commons Attribution-NonCommercial-NoDerivs 4.0 International License (CC BY-NC-ND 4.0), which permits the non-commercial replication and distribution of the article with the strict proviso that no changes or edits are made and the original work is properly cited (including links to both the formal publication through the relevant DOI and the license). See: https://creativecommons.org/licenses/by-nc-nd/4.0/.
References
- Casey JR, Grinstein S, Orlowski J. Sensors and regulators of intracellular pH. Nat Rev Mol Cell Biol 2010;11:50-61. [Crossref] [PubMed]
- Gatenby RA, Gawlinski ET, Gmitro AF, Kaylor B, Gillies RJ. Acid-mediated tumor invasion: a multidisciplinary study. Cancer Res 2006;66:5216-23. [Crossref] [PubMed]
- Webb BA, Chimenti M, Jacobson MP, Barber DL. Dysregulated pH: a perfect storm for cancer progression. Nat Rev Cancer 2011;11:671-7. [Crossref] [PubMed]
- Goldenberg JM, Pagel MD. Assessments of tumor metabolism with CEST MRI. NMR Biomed 2019;32:e3943. [Crossref] [PubMed]
- McVicar N, Li AX, Gonçalves DF, Bellyou M, Meakin SO, Prado MA, Bartha R. Quantitative tissue pH measurement during cerebral ischemia using amine and amide concentration-independent detection (AACID) with MRI. J Cereb Blood Flow Metab 2014;34:690-8. [Crossref] [PubMed]
- Pavuluri K, Manoli I, Pass A, Li Y, Vernon HJ, Venditti CP, McMahon MT. Noninvasive monitoring of chronic kidney disease using pH and perfusion imaging. Sci Adv 2019;5:eaaw8357. [Crossref] [PubMed]
- Longo DL, Cutrin JC, Michelotti F, Irrera P, Aime S. Noninvasive evaluation of renal pH homeostasis after ischemia reperfusion injury by CEST-MRI. NMR Biomed 2017; [Crossref] [PubMed]
- Zhang X, Lin Y, Gillies RJ. Tumor pH and its measurement. J Nucl Med 2010;51:1167-70. [Crossref] [PubMed]
- Chan KW, Liu G, Song X, Kim H, Yu T, Arifin DR, Gilad AA, Hanes J, Walczak P, van Zijl PC, Bulte JW, McMahon MT. MRI-detectable pH nanosensors incorporated into hydrogels for in vivo sensing of transplanted-cell viability. Nat Mater 2013;12:268-75. [Crossref] [PubMed]
- Mouffouk F, Serrai H, Bhaduri S, Achten R, Seyyedhamzeh M, Husain AA, Alhendal A, Zourob M. A New Class of Smart Gadolinium Contrast Agent for Tissue pH Probing Using Magnetic Resonance Imaging. Molecules 2020;25:1513. [Crossref] [PubMed]
- Raghunand N, Howison C, Sherry AD, Zhang S, Gillies RJ. Renal and systemic pH imaging by contrast-enhanced MRI. Magn Reson Med 2003;49:249-57. [Crossref] [PubMed]
- Garcia-Martin ML, Martinez GV, Raghunand N, Sherry AD, Zhang S, Gillies RJ. High resolution pH(e) imaging of rat glioma using pH-dependent relaxivity. Magn Reson Med 2006;55:309-15. [Crossref] [PubMed]
- Robey IF, Baggett BK, Kirkpatrick ND, Roe DJ, Dosescu J, Sloane BF, Hashim AI, Morse DL, Raghunand N, Gatenby RA, Gillies RJ. Bicarbonate increases tumor pH and inhibits spontaneous metastases. Cancer Res 2009;69:2260-8. [Crossref] [PubMed]
- Ward KM, Balaban RS. Determination of pH using water protons and chemical exchange dependent saturation transfer (CEST). Magn Reson Med 2000;44:799-802. [Crossref] [PubMed]
- Aime S, Calabi L, Biondi L, De Miranda M, Ghelli S, Paleari L, Rebaudengo C, Terreno E. Iopamidol: Exploring the potential use of a well-established x-ray contrast agent for MRI. Magn Reson Med 2005;53:830-4. [Crossref] [PubMed]
- Foo LS, Harston G, Mehndiratta A, Yap WS, Hum YC, Lai KW, Mohamed Mukari SA, Mohd Zaki F, Tee YK. Clinical translation of amide proton transfer (APT) MRI for ischemic stroke: a systematic review (2003-2020). Quant Imaging Med Surg 2021;11:3797-811. [Crossref] [PubMed]
- van Zijl PC, Yadav NN. Chemical exchange saturation transfer (CEST): what is in a name and what isn't? Magn Reson Med 2011;65:927-48. [Crossref] [PubMed]
- Liu G, Song X, Chan KW, McMahon MT. Nuts and bolts of chemical exchange saturation transfer MRI. NMR Biomed 2013;26:810-28. [Crossref] [PubMed]
- van Zijl PCM, Lam WW, Xu J, Knutsson L, Stanisz GJ. Magnetization Transfer Contrast and Chemical Exchange Saturation Transfer MRI. Features and analysis of the field-dependent saturation spectrum. Neuroimage 2018;168:222-41. [Crossref] [PubMed]
- Petroff OA, Prichard JW, Behar KL, Alger JR, Shulman RG. In vivo phosphorus nuclear magnetic resonance spectroscopy in status epilepticus. Ann Neurol 1984;16:169-77. [Crossref] [PubMed]
- Gillies RJ, Morse DL. In vivo magnetic resonance spectroscopy in cancer. Annu Rev Biomed Eng 2005;7:287-326. [Crossref] [PubMed]
- Gallagher FA, Kettunen MI, Day SE, Hu DE, Ardenkjaer-Larsen JH. Zandt Ri, Jensen PR, Karlsson M, Golman K, Lerche MH, Brindle KM. Magnetic resonance imaging of pH in vivo using hyperpolarized 13C-labelled bicarbonate. Nature 2008;453:940-3. [Crossref] [PubMed]
- Moon BF, Jones KM, Chen LQ, Liu P, Randtke EA, Howison CM, Pagel MD. A comparison of iopromide and iopamidol, two acidoCEST MRI contrast media that measure tumor extracellular pH. Contrast Media Mol Imaging 2015;10:446-55. [Crossref] [PubMed]
- Longo DL, Sun PZ, Consolino L, Michelotti FC, Uggeri F, Aime S. A general MRI-CEST ratiometric approach for pH imaging: demonstration of in vivo pH mapping with iobitridol. J Am Chem Soc 2014;136:14333-6. [Crossref] [PubMed]
- Yang X, Song X, Ray Banerjee S, Li Y, Byun Y, Liu G, Bhujwalla ZM, Pomper MG, McMahon MT. Developing imidazoles as CEST MRI pH sensors. Contrast Media Mol Imaging 2016;11:304-12. [Crossref] [PubMed]
- Sheth VR, Liu G, Li Y, Pagel MD. Improved pH measurements with a single PARACEST MRI contrast agent. Contrast Media Mol Imaging 2012;7:26-34. [Crossref] [PubMed]
- Liu G, Li Y, Sheth VR, Pagel MD. Imaging in vivo extracellular pH with a single paramagnetic chemical exchange saturation transfer magnetic resonance imaging contrast agent. Mol Imaging 2012;11:47-57. [Crossref] [PubMed]
- Thorarinsdottir AE, Du K, Collins JHP, Harris TD. Ratiometric pH Imaging with a CoII2 MRI Probe via CEST Effects of Opposing pH Dependences. J Am Chem Soc 2017;139:15836-47. [Crossref] [PubMed]
- Longo DL, Dastrù W, Digilio G, Keupp J, Langereis S, Lanzardo S, Prestigio S, Steinbach O, Terreno E, Uggeri F, Aime S. Iopamidol as a responsive MRI-chemical exchange saturation transfer contrast agent for pH mapping of kidneys: In vivo studies in mice at 7 T. Magn Reson Med 2011;65:202-11. [Crossref] [PubMed]
- Wu Y, Zhou IY, Igarashi T, Longo DL, Aime S, Sun PZ. A generalized ratiometric chemical exchange saturation transfer (CEST) MRI approach for mapping renal pH using iopamidol. Magn Reson Med 2018;79:1553-8. [Crossref] [PubMed]
- Terreno E, Castelli DD, Viale A, Aime S. Challenges for molecular magnetic resonance imaging. Chem Rev 2010;110:3019-42. [Crossref] [PubMed]
- Chen LQ, Howison CM, Jeffery JJ, Robey IF, Kuo PH, Pagel MD. Evaluations of extracellular pH within in vivo tumors using acidoCEST MRI. Magn Reson Med 2014;72:1408-17. [Crossref] [PubMed]
- Sun PZ, Longo DL, Hu W, Xiao G, Wu R. Quantification of iopamidol multi-site chemical exchange properties for ratiometric chemical exchange saturation transfer (CEST) imaging of pH. Phys Med Biol 2014;59:4493-504. [Crossref] [PubMed]
- Longo DL, Michelotti F, Consolino L, Bardini P, Digilio G, Xiao G, Sun PZ, Aime S. In Vitro and In Vivo Assessment of Nonionic Iodinated Radiographic Molecules as Chemical Exchange Saturation Transfer Magnetic Resonance Imaging Tumor Perfusion Agents. Invest Radiol 2016;51:155-62. [Crossref] [PubMed]
- Arena F, Irrera P, Consolino L, Colombo Serra S, Zaiss M, Longo DL. Flip-angle based ratiometric approach for pulsed CEST-MRI pH imaging. J Magn Reson 2018;287:1-9. [Crossref] [PubMed]
- Melkus G, Grabau M, Karampinos DC, Majumdar S. Ex vivo porcine model to measure pH dependence of chemical exchange saturation transfer effect of glycosaminoglycan in the intervertebral disc. Magn Reson Med 2014;71:1743-9. [Crossref] [PubMed]
- Ma X, Wang Y, Zhao T, Li Y, Su LC, Wang Z, Huang G, Sumer BD, Gao J. Ultra-pH-sensitive nanoprobe library with broad pH tunability and fluorescence emissions. J Am Chem Soc 2014;136:11085-92. [Crossref] [PubMed]
- Zaiss M, Schmitt B, Bachert P. Quantitative separation of CEST effect from magnetization transfer and spillover effects by Lorentzian-line-fit analysis of z-spectra. J Magn Reson 2011;211:149-55. [Crossref] [PubMed]
- Chen Z, Li Y, Airan R, Han Z, Xu J, Chan KWY, Xu Y, Bulte JWM, van Zijl PCM, McMahon MT, Zhou S, Liu G CT. Quant Imaging Med Surg 2019;9:1579-91. [Crossref] [PubMed]
- Cai K, Singh A, Poptani H, Li W, Yang S, Lu Y, Hariharan H, Zhou XJ, Reddy R. CEST signal at 2ppm (CEST@2ppm) from Z-spectral fitting correlates with creatine distribution in brain tumor. NMR Biomed 2015;28:1-8. [PubMed]
- Zhang Z, Zhang C, Yao J, Gao F, Gong T, Jiang S, Chen W, Zhou J, Wang G. Amide proton transfer-weighted magnetic resonance imaging of human brain aging at 3 Tesla. Quant Imaging Med Surg 2020;10:727-42. [Crossref] [PubMed]
- Jones KM, Randtke EA, Yoshimaru ES, Howison CM, Chalasani P, Klein RR, Chambers SK, Kuo PH, Pagel MD. Clinical Translation of Tumor Acidosis Measurements with AcidoCEST MRI. Mol Imaging Biol 2017;19:617-25. [Crossref] [PubMed]
- Müller-Lutz A, Khalil N, Schmitt B, Jellus V, Pentang G, Oeltzschner G, Antoch G, Lanzman RS, Wittsack HJ. Pilot study of Iopamidol-based quantitative pH imaging on a clinical 3T MR scanner. MAGMA 2014;27:477-85. [Crossref] [PubMed]