Amide proton transfer (APT) imaging-based study on the correlation between brain pH and voltage-gated proton channels in piglets after hypoxic-ischemic brain injury
Introduction
When the brain suffers hypoxic-ischemic (HI) injury, aerobic energy dysbolism occurs (1). Aerobic metabolism is replaced by anaerobic metabolism, and anaerobic metabolism results in the production of lactate (Lac). Subsequently, Lac accumulates in the brain (2,3). Lac accumulation leads to the suppression of glycometabolism and the depletion of adenosine triphosphate (ATP), which worsens intracellular acidosis (4). However, studies have shown that intracellular acidosis is not only caused by Lac accumulation but is also associated with nicotinamide adenine dinucleotide (NADH) accumulation and an increase in hydrogen ions (H+) in the brain (5), of which the increase in H+ is essential for a change in brain pH.
Under physiological conditions, the regulation of brain pH is important, and a normal pH is particularly critical for protein structure and enzymatic catalysis in the brain. The intracellular and extracellular pH ranges are approximately 7.2 to 7.3 and 7.3 to 7.4 in the mammalian brain, respectively (6,7). During cerebral anaerobic glycolysis after HI injury, Lac (C3H6O3) is produced from pyruvic acid (C3H4O3), in which a portion of H+ is consumed, and a portion is discharged to the intercellular space via ion channels and transporters.
Voltage-gated proton channels (Hv1) can regulate the pH in cells. Hv1 is pH sensitive and voltage-dependent and has a high selectivity to protons (8), thus hindering other cations from passing through it (9). In the central nervous system, Hv1 is mainly expressed in microglia (10). Hv1 can be activated by an intracellular acidic environment (11,12) and primarily mediates the outflow of H+. Such transport requires no consumption of ATP and is a type of passive transportation based on an electrochemical gradient.
Amide proton transfer (APT) imaging requires no exogenous contrast media. It is an imaging technique based on free proteins in the cells and the exchange between amide protons (in the polypeptides) and water protons. Specifically, amide protons are saturated using radio frequency (RF) pulses at a specified frequency and then exchanged with water protons. As a result, free water protons are partially saturated, and the MR signals of water are changed. Such a signal change depends on the exchange rate between the amide protons and water protons. The exchange rate is determined by protein concentration and pH in vivo (13). The most common APT quantitative analysis method is magnetization transfer ratio with asymmetrical analysis (MTRasym) at ±3.5 ppm. The MTR difference at ±3.5 ppm of the water resonance frequency is the APT signal intensity, and the latter mainly depends on the protein content and pH of cells. After HI injury, there is a decrease in brain pH, followed by a reduction in the exchange rate of amide protons and water protons in cells and a decline of APT signals (14,15).
This study aimed to investigate brain pH and Hv1 expression at different time points after HI injury. Furthermore, this study aimed to assess the shuttle of brain H+ in the neural network and changes in brain pH after HI injury.
Methods
Experimental animals
A total of 59 healthy piglets (age range, 3–5 days after birth; body weight, 1–1.5 kg; Yorkshire piglets or Large White piglets) were selected. Six piglets were excluded due to death or motion artifacts, leaving a total of 10 animals in the control group and 43 animals in the HI brain injury (HIBI) model group. Based on magnetic resonance imaging (MRI) scan time after HIBI, the model group was further divided into 6 subgroups (0–2 hours, n=8; 2–6 hours, n=8; 6–12 hours, n=6; 12–24 hours, n=10; 24–48 hours, n=5; and 48–72 hours, n=6).
Animal modeling
Control group
At a room temperature of 28 to 30 °C, 0.6 mL/kg Su-mian-xin (Changchun Military Veterinary Institute, Academy of Military Medical Science) was intramuscularly injected (16). Endotracheal intubation was performed with a tracheal cannula (diameter: 2.5 mm) for mechanical ventilation, and the tracheal cannula was connected to a TKR-200C small animal ventilator [Jiangxi Teli Anesthetic Ventilator Equipment (China) Co., Ltd.]. The ventilator parameters with 100% oxygen were as follows: inspiration/expiration ratio (I/E), 1:1.5; respiratory rate (RR), 30 bpm; and pressure, 0.05 to 0.06 MPa. A TuffSat hand-held pulse oximeter (GE, USA) was used to monitor the heart rate (HR) and pulse oxygen saturation (SpO2). Jugular catheterization was conducted, and then the catheter was fixed. After the neck skin was disinfected with iodophor, a median incision was made. Next, the bilateral common carotid arteries were dissected, and finally, the incision was sutured. The piglets were carefully encapsulated with thick quilts during the operation and placed into the incubator (912-005, Shenzhen Reward Life Technology Co., Ltd.) immediately after modeling, with the rectal temperature kept at 38 to 40 °C.
Model group
The same procedures outlined previously for the control group were performed on piglets in the model group. The common carotid arteries were clamped bilaterally with artery clips to restrict the blood flow, and this was maintained for 40 minutes by mechanical ventilation using 6% oxygen (Dalian Special Gases Co., Ltd.). Subsequently, 100% oxygen (Dalian Special Gases Co., Ltd.) was inhaled, and the blood supply of the bilateral common carotid arteries was restored simultaneously. Lastly, the incision was sutured. The SpO2 and HR were monitored during the entire process. Any episodes of shock or convulsions that occurred during and after the procedure were treated promptly. Mechanical ventilation was stopped after the recovery of spontaneous breathing. The piglets were placed into the incubator (912-005, Shenzhen reward Life Technology Co., Ltd.) immediately after modeling.
An MRI scan was performed in the model group at 0–2, 2–6, 6–12, 12–24, 24–48, and 48–72 hours after HI injury.
Except for imaging, the piglets were all fed in the incubator to maintain their body temperature and diet supply. The animals were encapsulated in thick quilts to ensure comfort and a constant body temperature during scanning.
APT scan and post-processing
MRI transverse scanning was performed using the Philips 3.0-T Achieva MRI system with 8-channel array coils. The scan parameters were as follows: time of repeat (TR), 3,000 ms; time of echo (TE), 7.5 ms; fast spin echo (FSE) factor, 54; saturation time at 2 µT, 800 ms; displacement, ±3.5 ppm; field of view (FOV), 170 mm × 145 mm; matrix, 108×71; and slice thickness, 5 mm. Simultaneous multi-slice coronal imaging was conducted for all piglets, with the basal ganglia oriented at the middle slice during scanning. APT images were analyzed and measured using interactive data language (IDL)-based software, and then their pseudo-color images were reconstructed. After the software automatically analyzed the raw data, the region of interest (ROI) (i.e., bilateral basal ganglia) was carefully marked in the obtained APT images by combining the conventional T2-weighted images and avoiding skull interference cerebrospinal fluid (CSF), and the cerebral ventricles. The APT value of the acquisition region reflected the signal intensity of the ROI.
Brain pH and histological examination
HIBI model piglets were sacrificed immediately after APT imaging, the skull was opened to expose the whole brain, then the bilateral cerebral hemispheres were dissected, and a pH electrode (Sartorius PB-10) was carefully inserted into the ROI (basal ganglia). Subsequently, the piglets were kept lying prostrate on the operation bench, and the pH electrode was vertically inserted 2.0 to 2.5 cm into the brain from the vertex (Figure 1). It was then withdrawn and inserted again into a new position in brain tissue for measurement. These measurements were repeated 4 times to obtain the pH in the bilateral basal ganglia and were completed as quickly as possible after animal sacrifice.
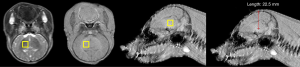
Histological examination and analysis
After the final MRI scan was finished, the cerebral hemispheres were rapidly collected and fixed in 10% neutral formalin for 24 to 48 hours. Next, the tissues were coronally cut into 4-mm sections, but the slices containing the basal ganglia and hippocampus were preserved. The tissue sections were subject to Hv1 immunofluorescent staining (Hv1 polyclonal antibody, Abcam, ab117520). The images were acquired under the confocal microscope (ZEISS LSM880).
Evaluation and processing of experimental results
Hv1 immunofluorescent staining results were evaluated by two pathologists (during their analysis, the pathologists were blinded to the identity of the samples, and they were unaware of grouping information). The immunohistochemical images were analyzed for optical density (OD) and observed under the microscope [high power (HP) ×400]. A greater OD indicated higher Hv1 expression, and Hv1 was expressed in the cell membrane of microglia. These indicators were observed in 5 fields of the basal ganglia, and the data obtained in these 5 fields were used to analyze and evaluate Hv1 expression within each group at different time points. The relative grayscale was analyzed with Image J analysis software.
Statistical analysis
SPSS software for Windows (version 22.0, Chicago, IL, USA) was used for the statistical processing of data. The quantitative data were presented as mean ± standard deviation (SD). Before ANOVA, the data were analyzed for homogeneity of variance using the Levene test, and the normal distribution and homogeneity of the variance of data in each group were validated. One-way ANOVA analyzed the data showing homogeneity of variance, and the intergroup comparison of the mean was performed using LSD t-testing. The correlations among APT value, Hv1 expression, and pH were investigated by Spearman rank correlation analysis, and a P value less than 0.05 was considered a statistically significant difference.
Experiments were performed under a project license (“Regulations for the Administration of Affairs Concerning Experimental Animals” and “Measures for the Administration of Licenses Concerning Experimental Animals”, No. 2015PS337K) granted by the China Medical University ethics committee, in compliance with the China Medical University guidelines for the care and use of animals.
Results
APT values in the basal ganglia at different time points after HIBI
There was no significant difference in the APT value of the basal ganglia between the left and right cerebral hemispheres (mean ± SD: left, 0.53±0.089; right, 0.54±0.092; P=0.82). Thus, the mean APT value of the bilateral basal ganglia was included in each group’s analysis. As shown by the statistical results of the APT value, there was no significant difference between the 24–48 or 48–72 hours HIBI model subgroups and the control group (P=0.094 and P=0.341, respectively). However, a significant difference between the remaining HIBI model subgroups and the control group was noted (control vs. 0–2 hours, P<0.001; control vs. 2–6 hours, P<0.001; control vs. 6–12 hours, P<0.001; control vs. 12–24 hours, P<0.001) (Figure 2).
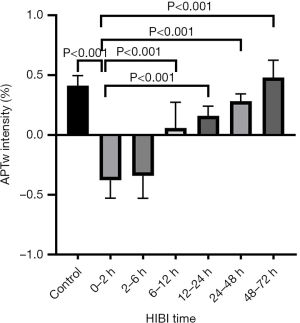
Hv1 expression in the basal ganglia and pH measurements at different time points after HIBI
In terms of Hv1 expression, there was a significant difference between the control group and the HIBI model subgroups (0–2, 2–6, 6–12, and 12–24 hours, P=0.002, P<0.001, P=0.005, and P=0.03, respectively), except for the 24–48 and 48–72 hours subgroups (P=0.628 and P=0.438, respectively). Hv1 expression reached a peak at 2–6 hours, and there was a significant difference between the control group and all HIBI model subgroups (P<0.001) (Figures 3,4).
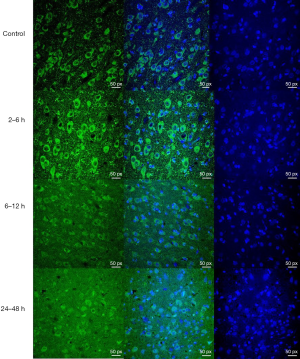
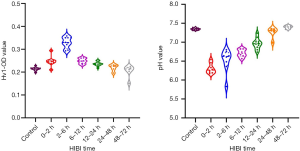
A statistically significant difference in pH was observed between the control group and all HIBI model subgroups (P<0.001), excluding the 24–48 and 48–72 hours subgroups (P=0.17 and P=0.38, respectively).
Correlations among the APT value of the basal ganglia, Hv1 expression, and pH at different time points after HIBI
After HI injury, the APT value of the basal ganglia had a significant negative correlation with Hv1 expression (ρ=–0.727; P<0.001), but a linear positive correlation with pH (ρ=0.862; P<0.001). There was a significant negative correlation between pH and Hv1 expression (ρ=–0.666; P<0.001) (Figure 5).
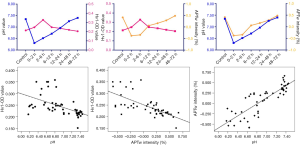
Discussion
The brain of neonates is under continuous development and maturation and has a great demand for oxygen. Under normal physiological conditions, the internal environment of the brain is in a steady state. When HI injury occurs, there is intracellular energy dysbolism, and the energy produced by aerobic metabolism is insufficient; thus, anaerobic metabolism occurs, which causes cerebral acidosis (17). HI, injury-induced acidosis is associated with many factors, such as Lac accumulation, NADH accumulation, and a predominant increase in H+. The pH gradient is associated with the mode of energy metabolism and the self-regulation of specific proteins. Our previous study results have suggested that astrocyte (AS) swelling may occur early after HI injury (18). Cell swelling and energy depletion after HI injury cause a failure of the ion pump on the cell membrane surface, resulting in deionization. This leads to the opening of different ion channels and a resultant change in the number of intracellular and extracellular H+.
Hv1 has a high selectivity for H+ (19) and mainly mediates H+ outflow based on the electrochemical gradient, which is a type of passive transportation. Hv1 is primarily expressed in microglia. There are many microglia in the brain, including all encephalic regions and gray and white matter (20). The Hv1 antibody (ab117520, whose specificity was only confirmed in the human body) was used in this study. Since Hv1 antibodies used in pigs on the market are very rare and the protein gene similarity between pigs and humans is very high, we used this antibody to react with pigs. Fortunately, our results showed that the Hv1 protein was expressed in microglial cells. In the follow-up study, this aspect will be improved. After HI injury, microglia cells are among the earliest to respond. Their activation can result in the production of numerous oxygen radicals, and these radicals can react with lipids, proteins, coenzyme factors, and DNA. Therefore, microglia are capable of inducing neuron or gliocyte injury and apoptosis (21,22). This study showed that after HIBI, Lac was produced in the cells via anaerobic glycolysis and ATP hydrolysis resulted in the massive release of protons. This was followed by a decrease in pH and an increase in Hv1 protein expression. We presumed that this occurred because of the intracellular acidosis that resulted after HIBI, and acidification of the intracellular environment led to increased Hv1 expression. If the concentration of H+ in the cells exceeded the buffering capability of the cells, H+ was discharged from cells via expressed Hv1. Therefore, increased Hv1 expression resulted from the increase in protons, and both variables were positively correlated, and the extracellular increase in H+ led to a concomitant decrease in extracellular pH (to ≤6.5) (5). The pH electrode was used to measure extracellular pH in the brain. After HI, protons within the cells increased and were released from the cells by increasingly expressed Hv1. As a result, extracellular pH as measured in the brain reflected the intracellular pH level.
This decrease in extracellular pH activated acid-sensitive ion channels (ASICs) and the inflow of sodium ions (Na+) and calcium ions (Ca2+) (23). The cells became depolarized, thus activating voltage-gated Ca2+ channels and N-methyl-D-aspartic acid (NMDA) glutamate (Glu) receptors, which resulted in further Ca2+ overload in numerous cells and secondary neuronal injury (24,25). Normally, the release, uptake, and reabsorption of Glu are in a dynamic balance. After HI injury, however, the increased release or injured reabsorption mechanism of Glu causes a sharp elevation of extracellular Glu level, thereby activating the NMDA receptor. The activation of NMDA receptors can induce neuronal excitotoxicity (26-28), which requires a release of superoxide via nicotinamide adenine dinucleotide phosphate (NADPH) oxidase in the neurons (29). The pH and Na+/H+ exchange in neurons are effective regulators for the production of excitatory superoxide (30).
Some studies have shown that mild acidosis can protect nerves injured by excitotoxicity and ischemia-reperfusion (31-34). Active oxygen produced by microglia in Hv1 knockout mice is significantly less than that in wild-type mice, with a decrease in activated microglia and apoptotic neurons (35,36). The reason for this is that only a minimal decline in intracellular pH leads to the activation and resultant uncoupling of NADPH oxidase and the NMDA receptor in neurons, thus preventing neuronal apoptosis. Therefore, the regulation of brain pH is critical for brain survival after HI injury (6). However, cerebral acidosis occurs after HIBI, and Hv1 can promote the outflow of H+ in the cells, thus mitigating intracellular acidosis and stabilizing the intracellular environment. In this way, Hv1 expression in microglia is a “double-edged sword” (meaning it has positive and negative effects) for neonates after HI injury. These findings support an association between metabolic activity and excitotoxicity. After HIBI, pH was first decreased and then reached a trough at 0–2 hours, whereas Hv1 expression was increased and peaked at 2–6 hours, which shows a nearly identical change. The high expression of Hv1 promoted the outflow of excessive H+ from within the cells to the extracellular space. Then the H+ produced by anaerobic metabolism were discharged with the recovery of aerobic metabolism and blood flow, followed by the recovery of pH, which eventually resulted in an associated reduction in Hv1 expression. Meanwhile, HIBI induced the injury and necrosis of partial nerve cells, which resulted in an associated decrease in Hv1 expression.
Because the coupling mechanism of activated NMDA receptors and superoxidase is associated with pH and Na+/H+ exchange in neurons, it can be inferred that interfering with H+ protein channels in this time window can influence brain pH to a certain degree, and therefore delay acidosis-induced brain injury after HIBI.
After HI injury, APT signal intensity first decreased and then increased, showing a changing trend coincident with brain pH. The decrease in APT value is secondary to intracellular acidosis caused by anaerobic metabolism. The subsequent increase in APT value is due to the increase in brain pH due to the recovery of aerobic metabolism and the excretion of H+ by reperfusion. This explanation is based on the hypothesis that the protein concentration in the brain remains unchanged after HI injury, and APT signal intensity is positively correlated with brain pH.
Conclusions
After HIBI, the pathophysiological processes are extremely complex, involving intracellular and extracellular ion steady states and abnormal electrical activities of cells, among other factors. Hv1 protein expression mutually influences and regulates intracellular pH change and energy metabolism in the brain.
Acknowledgments
Funding: This work was supported by the National Natural Science Foundation of China (No. 81871408), National Science Foundation for Young Scientists of China (No. 81801658), Outstanding Scientific Fund of Shengjing Hospital (No. 201402), and 345 Talent Project of Shengjing Hospital.
Footnote
Conflicts of Interest: Both authors have completed the ICMJE uniform disclosure form (available at https://dx.doi.org/10.21037/qims-21-250). The authors have no conflicts of interest to declare.
Ethical Statement: The authors are accountable for all aspects of the work in ensuring that questions related to the accuracy or integrity of any part of the work are appropriately investigated and resolved. Experiments were performed under a project license (“Regulations for the Administration of Affairs Concerning Experimental Animals” and “Measures for the Administration of Licenses Concerning Experimental Animals”, No. 2015PS337K) granted by the China Medical University ethics committee, in compliance with the China Medical University guidelines for the care and use of animals.
Open Access Statement: This is an Open Access article distributed in accordance with the Creative Commons Attribution-NonCommercial-NoDerivs 4.0 International License (CC BY-NC-ND 4.0), which permits the non-commercial replication and distribution of the article with the strict proviso that no changes or edits are made and the original work is properly cited (including links to both the formal publication through the relevant DOI and the license). See: https://creativecommons.org/licenses/by-nc-nd/4.0/.
References
- Greco P, Nencini G, Piva I, Scioscia M, Volta CA, Spadaro S, Neri M, Bonaccorsi G, Greco F, Cocco I, Sorrentino F, D'Antonio F, Nappi L. Pathophysiology of hypoxic-ischemic encephalopathy: a review of the past and a view on the future. Acta Neurol Belg 2020;120:277-88. [Crossref] [PubMed]
- Zheng Y, Wang XM. Measurement of Lactate Content and Amide Proton Transfer Values in the Basal Ganglia of a Neonatal Piglet Hypoxic-Ischemic Brain Injury Model Using MRI. AJNR Am J Neuroradiol 2017;38:827-34. [Crossref] [PubMed]
- Khong PL, Tse C, Wong IY, Lam BC, Cheung PT, Goh WH, Kwong NS, Ooi GC. Diffusion-weighted imaging and proton magnetic resonance spectroscopy in perinatal hypoxic-ischemic encephalopathy: association with neuromotor outcome at 18 months of age. J Child Neurol 2004;19:872-81. [Crossref] [PubMed]
- Distefano G, Pratico AD. Actualities on molecular pathogenesis and repairing processes of cerebral damage in perinatal hypoxic-ischemic encephalopathy. Ital J Pediatr 2010;36:63. [Crossref] [PubMed]
- Vannucci RC. Experimental biology of cerebral hypoxia-ischemia: relation to perinatal brain damage. Pediatr Res 1990;27:317-26. [Crossref] [PubMed]
- Uria-Avellanal C, Robertson NJ. Na(+)/H(+) exchangers and intracellular pH in perinatal brain injury. Transl Stroke Res 2014;5:79-98. [Crossref] [PubMed]
- Casey JR, Grinstein S, Orlowski J. Sensors and regulators of intracellular pH. Nat Rev Mol Cell Biol 2010;11:50-61. [Crossref] [PubMed]
- DeCoursey TE. Voltage and pH sensing by the voltage-gated proton channel, HV1. J R Soc Interface 2018;15:20180108 [Crossref] [PubMed]
- Schladt TM, Berger TK. Voltage and pH difference across the membrane control the S4 voltage-sensor motion of the Hv1 proton channel. Sci Rep 2020;10:21293. [Crossref] [PubMed]
- Wu LJ. Microglial voltage-gated proton channel Hv1 in ischemic stroke. Transl Stroke Res 2014;5:99-108. [Crossref] [PubMed]
- Meitzler JL, Antony S, Wu Y, Juhasz A, Liu H, Jiang G, Lu J, Roy K, Doroshow JH. NADPH oxidases: a perspective on reactive oxygen species production in tumor biology. Antioxid Redox Signal 2014;20:2873-89. [Crossref] [PubMed]
- DeCoursey TE, Hosler J. Philosophy of voltage-gated proton channels. J R Soc Interface 2013;11:20130799 [Crossref] [PubMed]
- Zhou J, Payen JF, Wilson DA, Traystman RJ, van Zijl PC. Using the amide proton signals of intracellular proteins and peptides to detect pH effects in MRI. Nat Med 2003;9:1085-90. [Crossref] [PubMed]
- Zhou J, Yan K, Zhu H. A simple model for understanding the origin of the amide proton transfer MRI signal in tissue. Appl Magn Reson 2012;42:393-402. [Crossref] [PubMed]
- Zheng Y, Wang X. The Applicability of Amide Proton Transfer Imaging in the Nervous System: Focus on Hypoxic-Ischemic Encephalopathy in the Neonate. Cell Mol Neurobiol 2018;38:797-807. [Crossref] [PubMed]
- Wang XY, Wang HW, Fu XH, Zhang WQ, Wu XY, Guo QY, Wang XM. Expression of N-methyl-d-aspartate receptor 1 and its phosphorylated state in basal ganglia of a neonatal piglet hypoxic-ischemic brain injury model: a controlled study of (1)H MRS. Eur J Paediatr Neurol 2012;16:492-500. [Crossref] [PubMed]
- Rainaldi MA, Perlman JM. Pathophysiology of Birth Asphyxia. Clin Perinatol 2016;43:409-22. [Crossref] [PubMed]
- Walz W, Klimaszewski A, Paterson IA. Glial swelling in ischemia: a hypothesis. Dev Neurosci 1993;15:216-25. [Crossref] [PubMed]
- DeCoursey TE. Voltage-gated proton channels: what's next? J Physiol 2008;586:5305-24. [Crossref] [PubMed]
- Chew LJ, Takanohashi A, Bell M. Microglia and inflammation: impact on developmental brain injuries. Ment Retard Dev Disabil Res Rev 2006;12:105-12. [Crossref] [PubMed]
- Mallard C, Tremblay ME, Vexler ZS. Microglia and Neonatal Brain Injury. Neuroscience 2019;405:68-76. [Crossref] [PubMed]
- Lv Y, Sun B, Lu XX, Liu YL, Li M, Xu LX, Feng CX, Ding X, Feng X. The role of microglia mediated pyroptosis in neonatal hypoxic-ischemic brain damage. Biochem Biophys Res Commun 2020;521:933-8. [Crossref] [PubMed]
- Waldmann R, Champigny G, Bassilana F, Heurteaux C, Lazdunski M. A proton-gated cation channel involved in acid-sensing. Nature 1997;386:173-7. [Crossref] [PubMed]
- Gao S, Yu Y, Ma ZY, Sun H, Zhang YL, Wang XT, Wang C, Fan WM, Zheng QY, Ma CL. NMDAR-Mediated Hippocampal Neuronal Death is Exacerbated by Activities of ASIC1a. Neurotox Res 2015;28:122-37. [Crossref] [PubMed]
- Herrera Y, Katnik C, Rodriguez JD, Hall AA, Willing A, Pennypacker KR, Cuevas J. sigma-1 receptor modulation of acid-sensing ion channel a (ASIC1a) and ASIC1a-induced Ca2+ influx in rat cortical neurons. J Pharmacol Exp Ther 2008;327:491-502. [Crossref] [PubMed]
- Cortey A. Cerebral hypoxic and ischemic damage in newborn infants: cellular mechanisms and role of excitatory amino acids. Arch Pediatr 1995;2:1192-9. [Crossref] [PubMed]
- Rego AC, Santos MS, Oliveira CR. Oxidative stress, hypoxia, and ischemia-like conditions increase the release of endogenous amino acids by distinct mechanisms in cultured retinal cells. J Neurochem 1996;66:2506-16. [Crossref] [PubMed]
- Dang YX, Shi KN, Wang XM. Early Changes in Glutamate Metabolism and Perfusion in Basal Ganglia following Hypoxia-Ischemia in Neonatal Piglets: A Multi-Sequence 3.0T MR Study. Front Physiol 2017;8:237. [Crossref] [PubMed]
- Minnella AM, Zhao JX, Jiang X, Jakobsen E, Lu F, Wu L, El-Benna J, Gray JA, Swanson RA. Excitotoxic superoxide production and neuronal death require both ionotropic and non-ionotropic NMDA receptor signaling. Sci Rep 2018;8:17522. [Crossref] [PubMed]
- Wang Q, Lv H, Lu L, Ren P, Li L. Neonatal hypoxic-ischemic encephalopathy: emerging therapeutic strategies based on pathophysiologic phases of the injury. J Matern Fetal Neonatal Med 2019;32:3685-92. [Crossref] [PubMed]
- Lam TI, Brennan-Minnella AM, Won SJ, Shen Y, Hefner C, Shi Y, Sun D, Swanson RA. Intracellular pH reduction prevents excitotoxic and ischemic neuronal death by inhibiting NADPH oxidase. Proc Natl Acad Sci U S A 2013;110:E4362-8. [Crossref] [PubMed]
- Li Y, Ritzel RM, He J, Cao T, Sabirzhanov B, Li H, Liu S, Wu LJ, Wu J. The voltage-gated proton channel Hv1 plays a detrimental role in contusion spinal cord injury via extracellular acidosis-mediated neuroinflammation. Brain Behav Immun 2021;91:267-83. [Crossref] [PubMed]
- Ritzel RM, He J, Li Y, Cao T, Khan N, Shim B, Sabirzhanov B, Aubrecht T, Stoica BA, Faden AI, Wu LJ, Wu J. Proton extrusion during oxidative burst in microglia exacerbates pathological acidosis following traumatic brain injury. Glia 2021;69:746-64. [Crossref] [PubMed]
- Li W, Ward R, Dong G, Ergul A, O'Connor P. Neurovascular protection in voltage-gated proton channel Hv1 knock-out rats after ischemic stroke: interaction with Na+ /H+ exchanger-1 antagonism. Physiol Rep 2019;7:e14142 [Crossref] [PubMed]
- Wu LJ, Wu G, Akhavan Sharif MR, Baker A, Jia Y, Fahey FH, Luo HR, Feener EP, Clapham DE. The voltage-gated proton channel Hv1 enhances brain damage from ischemic stroke. Nat Neurosci 2012;15:565-73. [Crossref] [PubMed]
- Yu Y, Luo X, Li C, Ding F, Wang M, Xie M, Yu Z, Ransom BR, Wang W. Microglial Hv1 proton channels promote white matter injuries after chronic hypoperfusion in mice. J Neurochem. 2020;152:350-67. [Crossref] [PubMed]