A novel wireless optical technique for quantitative evaluation of cerebral perfusion pressure in a fluid percussion animal model of traumatic brain injury
Introduction
Traumatic brain injuries (TBIs) are usually caused by falls, vehicle accidents, and assault, and result in significant death and disability worldwide. The outcome of TBIs can be divided into primary and secondary brain injury. Primary brain injury occurs at the moment external forces, such as direct collision, acceleration or deceleration, or rotational forces, are exerted on the brain. Since primary brain injury occurs so rapidly, it is difficult to prevent and probably leads to secondary brain injury with delayed injury occurring in the hours and days following the initial brain injury. The major causes of secondary brain injury include ischemia, cerebral hypoxia, and increased intracranial pressure (ICP). Therefore, the main scope of multimodality management of TBI patients admitted to the intensive care unit is to obtain a continuous multiparameter monitoring designed to minimize secondary brain injury which could lead to clinical worsening and need for surgical intervention (1-4).
Cerebral perfusion pressure (CPP) monitoring has become the gold standard in clinical practice to prevent secondary brain injury in patients with TBI. CPP, defined as the difference between mean arterial pressure (MAP) and ICP, has been reported to be associated with cerebral blood flow (CBF), and the value of CPP in critical care has been reported to indirectly reflect whether there is sufficient oxygenated blood flow into the brain. After a TBI, increased ICP causing lower CPP is the main cause of cerebral ischemia, which leads to cerebral hypoxia and has been proven to be a trigger of secondary brain injury (5). Partial pressure of brain tissue oxygen (PbtO2) monitoring is often used to evaluate cerebral oxygenation levels, and a relationship between CPP and cerebral oxygenation has been proven in previous studies. In 2005, Jaeger et al. showed a relationship between CPP, regional cerebral blood flow (rCBF), and PbtO2 after TBI, and the change in CPP was the same as that seen in rCBF and PbtO2 (6), and in 2011, Friess et al. found a strong correlation between CPP and PbtO2 after brain injury in swine (7). However, PbtO2 monitoring is an invasive process that may increase the risk of brain hemorrhage and infection, and is not convenient in clinical practice (8).
Near-infrared spectroscopy (NIRS) is widely used in neurology to assess cerebral ischemia and cerebral hypoxia. In 1985, Ferrari et al. first proposed applying NIRS to human brains (9). Some pioneers indicated that NIRS could check the pathophysiology of brain injury though monitoring cerebral oxygenation and hemodynamics in diseased infants (10). NIRS has several advantages over PbtO2, including the ability to demonstrate global cerebral oxygenation, and that it is non-invasive. To address the unmet needs of TBI management the neurotraumatology community has now focused on NIRS to monitor cerebral perfusion and brain permeability (11,12) In this study, a wireless multi-channel NIRS was use to record continuous concentration changes of oxy-hemoglobin (HbO2), deoxy-hemoglobin (HbR), and total-hemoglobin (HbT) of the brain during and after TBI. Changes in CPP (∆CPP) during TBI were also monitored. The relationships between HbO2, HbR and HbT, and CPP under different traumatic intensity were analyzed.
Methods
NIRS system
A NIRS system (Figure 1) was designed and implemented to continuously monitor the relative concentrations of HbO2 and HbR under TBI (13). The NIRS probe consisted of light emitting diodes (LEDs) (SMT735/850, EPITEX, Japan) and photodiodes (PDs) (PD15-22C/TR8, EVERLIGHT, Taiwan). The LEDs were used to provide 735 nm and 850 nm wavelength light sources, and the PDs were used to detect and convert the diffusely reflected lights into electrical signals. Most of the light emitted by the LEDs will be rapidly diffused when passing through biological tissue due to scattering, and only part of the light will be absorbed by the tissue, especially HbO2 and HbR, which are the main absorbers of near-infrared wavelength light. The diffusely reflected light detected by the PDs will be delivered to the wireless signal acquisition module, then amplified, filtered, digitized, and transmitted wirelessly to the host system. The power of the light source is less than 3 mW, and the sampling rate of this system is set to 25 Hz. The wireless options are particularly designed for a further awake animal model of NIRS cerebral perfusion experiment.
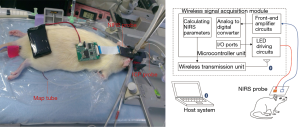
In general, the mean path of light is shaped like a banana, and the maximum penetrating depth is about a half of the distance between the light source and the detector (14,15). By using this rule, the specific monitoring area can be determined. The variation in the concentrations of HbO2 and HbR can be quantified by modifying the Beer-Lambert law. For a specific wavelength λ, the variation in the concentration of absorber, such as ∆[HbO2] and ∆[HbT], will cause the change of optical intensity
where If and Ii denote the reflected light intensities before and after the concentration change respectively, B is the differential path length factor, and L denotes the distance between the light source and the detector. Here, and denote the extinction coefficients of HbO2 and HbT respectively. Next, the variations in the concentrations of HbO2 and HbT can be solved by using the change of the optical intensity corresponding to two or more different wavelengths
and the changes in total-hemoglobin can be defined as:
Animal preparation
Adult male Sprague-Dawley rats (n=16), weighing 375±25 g were used in the experiments. All rats were raised on a 12-hour light/12-hour dark cycle and allowed free access to food and water. All experimental procedures conformed to the guidelines of the National Institute of Health and were approved by the Animal Care and Use Committee of Chi-Mei Medical Center (Affidavit of approval of Animal Use Protocol, Chi-Mei medical center, IACUC Approval No. 102120603) to minimize discomfort to the animals during the surgery and recovery periods. The 16 rats were randomly assigned to four impact groups (sham, 1.6, 2.0, and 2.4 atm), and monitored by the wireless NIRS system, and ICP and MAP devices. The rats were anesthetized with sodium pentothal (25 mg/kg, i.p.; Sigma Chemical Co., St Louis, MO, USA) and a mixture containing ketamine (44 mg/kg, i.m.; Nan Kuang Pharmaceutical, Tainan, Taiwan), atropine (0.02633 mg/kg, i.m.; Sintong Chemical Industrial Co., Ltd., Taoyuan, Taiwan), and xylazine (6.77 mg /kg, i.m.; Bayer, Leverkusen, Germany), and were sacrificed on the third day post-surgery.
Experiment design for TBI
In this study, a fluid percussion injury (FPI) model was used to reproduce TBI in the rats (16). The rats were first anesthetized and their heads then placed into a stereotaxic frame, and the ears were inserted using ear bars to secure the head. To maintain the core temperature at 37 °C, a rectal temperature probe attached to a thermostatic controller was inserted into the colon. The head fur was then trimmed, and the scalp sagittally incised. A circular craniotomy on the skull was drilled to set the impact point, which was located anterior-posteriorly −3 mm and laterally +4 mm from the bregma and a luer-lock connector with a sealed and fluid-filled reservoir was secured into the craniotomy. The wireless NIRS system, and ICP and MAP monitoring devices were then installed and monitoring was performed for two hours. A pendulum then struck the reservoir to generate a fluid wave to impact the brain, and a respiratory treatment procedure was carried out to assist the respiration of the rats immediately after the FPI experiment. Upon completion of the experiments, the monitoring devices and connector were removed, and the incisions were sutured.
The monitoring position of the NIRS probe focused on the striatum region of the brain, and the maximum penetrating depth was approximately 8 mm and located anterior-posteriorly −0.5 mm and laterally +3.5 mm from the bregma. The changes in 30-second HbO2 and HbR were recorded as the data baseline before the FPI experiments.
The mean arterial blood pressure was measured by cannulating the femoral artery with a 3-way stopcock connected with a pressure transducer and a data acquisition system (PowerLab/8sp, ADInstruments) was also used. In addition, a pressure-monitoring catheter to monitor ICP (Codman ICP Express Monitor, Codman & Shurtleff, Inc.) inserted anterior-posteriorly −0.8 mm and laterally +4 mm from the bregma, was installed on the stereotaxic frame. When the experiment began, the MAP and ICP data were initially recorded as the baseline. Thereafter, MAP and ICP values were recorded per 10 minutes. For recording the short-term MAP and ICP, the data during impact and 4.5 minutes after impact were also recorded (Supplementary file).
Statistical analysis
The time courses of HbO2, HbR, HbT, CPP, and ICP at different impact strengths, and their mean values after FPI at different impact strengths were analyzed using analysis of variance. Power analysis is listed in Table 1. Significance was defined as a P value of less than 0.05 and the linear correlation coefficients between CPP and NIRS parameters were analyzed using MATLAB.
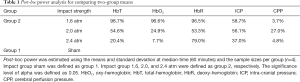
Full table
Results
The changes in relative concentrations of HbO2 {∆[HbO2]}, HbR {∆[HbR]}, HbT {∆[HbT]}, CPP, and ICP during and after TBI were first investigated. Bland–Altman analysis was performed first, showing most of the measured differences within the limit of agreement (Figure 2) and the concentration changes ∆[HbO2], ∆[HbR], and ∆[HbT] during and after the different impact strengths are shown in Figure 3. The time of the impact point was set at 0.5 minutes, and the respiratory treatment was carried out within 1 minute after the impact to help with spontaneous respiration. The experimental results showed that ∆[HbO2], ∆[HbR], and ∆[HbT] dropped immediately at the impact point and then rose rapidly after the respiratory treatment. The trends of ∆[HbO2], ∆[HbR], and ∆[HbT] were inversed to the increased impact intensity. Moreover, ∆[HbO2] and ∆[HbT] corresponded to the different impact intensity significantly. While after the FPI experiments, ∆[HbO2], ∆[HbR], and ∆[HbT] were higher than the baseline values, only ∆[HbO2] at an impact strength of 2.4 atm was lower than the baseline value.
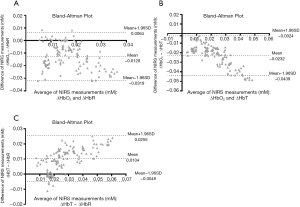
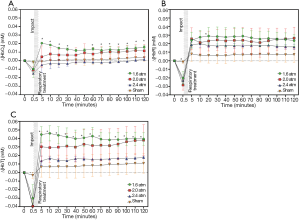
The time courses of ∆ICP and ∆CPP corresponding to different impact intensities are shown in Figures 4,5,respectively. Increasing impact strengths led to an increase in ∆ICP. Moreover, the trend of change in ∆CPP corresponding to different impact strengths was like that of ∆[HbO2], ∆[HbR], and ∆[HbT]. For an impact strength of 2.4 atm, ∆CPP after TBI was almost lower than its baseline value. Table 2 shows the results of the average and standard deviation of ∆CPP, ∆[HbR], ∆[HbO2], and ∆[HbT] after TBI corresponding to different impact strengths and reveals the values of ∆CPP, ∆[HbR], ∆[HbO2], and ∆[HbT] decreased with increasing impacts.
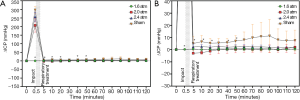
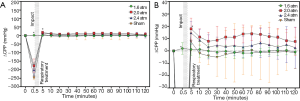

Full table
The correlations between ∆CPP, and ∆[HbO2], ∆[HbR], and ∆[HbT] are shown in Figure 6. The relation between both ∆CPP and ∆[HbO2] (correlation =0.72) and ∆[HbT] (correlation =0.6) were significant (correlation =0.72), and the correlation between ∆CPP and ∆[HbR] was lower (correlation =0.36).

Discussion
According to the results (Figures 2,3A), ∆[HbO2], ∆[HbR], and ∆[HbT] and ∆CPPs dropped immediately at the impact point and the ∆ICP rose rapidly. This can be explained by the post-FPI apnea causing cerebral hypoxia and the distortion or displacement of the cerebral vasculature causing an increased ICP leading to a decrease in cerebral flow and cerebral hypoperfusion. The increased strength of trauma was associated with increasing ∆ICP (17) and decreasing ∆CPP at the impact point (Figures 3A,4A, respectively).
Cerebral hyperperfusion can occur in the early stage of TBI (17), and this was seen in our ∆CPP results (Figure 4B) where cerebral hyperperfusion occurred immediately after the TBI. Likewise, hyperemia following post-traumatic ischemia has been reported to occur due to metabolic demand by effective autoregulation (18), and this was also reflected in ∆[HbO2], ∆[HbR], and ∆[HbT] after the FPI experiments in this study (Figure 2). At 1.6 to 2.4 atm of impact strength, the increasing ∆ICP corresponded to decreases in ∆CPP, ∆[HbO2], ∆[HbR], and ∆[HbT] (Figure 3B and Table 1). This can be explained by an impairment in cerebral autoregulation, which saw the increasing ICP cause cerebral hypoperfusion (19,20) leading to a decrease in cerebral blood volume (21) and cerebral oxygenation (22), especially at 2.4 atm of impact strength. The values of ∆[HbR] were all higher than ∆[HbO2] at 1.6, 2.0, and 2.4 atm. This can be explained by the injured brain cells needing more oxygenated hemoglobin to metabolize (23). Stocchetti et al. reported that increased CPP was positively correlated to cerebral oxygenation as it provides more oxygenated blood flow into the brain (24), and this is consistent with the present findings in the correlation between ∆CPP and ∆[HbO2] (Figure 5A).The increases in ∆CPP correlates with increases in brain oxygenation immediately after impact but may also imply disturbed autoregulation. The increase in CPP represents greater blood flow into the brain so the ∆CPP was also positively correlated to ∆[HbT]. The correlation between ∆CPP and ∆[HbR] was lower because deoxy-hemoglobin is associated with cell metabolism. The more injured brain cells require oxygen to metabolize, the more deoxy-hemoglobin is produced. Forcione et al. have nicely shown how this can be done with dynamic contrast-enhanced NIRS with indocyanine green in TBI patients (25,26). The manner by which CBF can be measured has been established, including intravascular assessment, nuclear medicine (133Xe, 85Kr, and PET), thermodilution, ultrasound, and biomedical optics (27,28). However, this is indirect evidence as ∆[HbT] and ∆CPP, are not identical to cerebral flow increase (29). Further survey is mandatory to clarify the relationship among the CBF, autoregulation, and NIRS parameters for applying this tool in clinical scenarios.
Study limitation
The major limitation of this study is its small sample size. We also lack direct measurement of the CBF in different CPP, which may decrease the originality of the study. The overall correlation between CPP and NIRS parameters is not high, possibly because the CPP may also be impacted by variation of ICP after brain trauma. Any experimental study on TBI is as good as the animal model used to test a research hypothesis and in our study a fluid percussion model seemed appropriate for the qualitative evaluation of CPP with NIRS. However further experiments on different injury models are warranted to confirm our findings.
Conclusions
The results showed that ∆CPP and ∆[HbO2], ∆[HbR], and ∆[HbT] were inversed to increased impact intensities. This data could be explained by the increase in ICP owing to increased impact intensities obstructing sufficient blood flow into the brain. In addition, ∆[HbO2] and ∆[HbT] were correlated to ∆CPP positively. These results favor that the NIRS system may potentially assess cerebral ischemia and oxygenation non-invasively, and that changes of HbO2 and HbT may be used to assess the level of CPP.
Acknowledgments
This manuscript was edited by QIMS English editing services.
Funding: This research was partly supported by the Ministry of Science and Technology in Taiwan, under grants MOST 108-2221-E-009-054-MY2. This research was also partly supported by the Higher Education Sprout Project of the National Chiao Tung University and Ministry of Education (MOE), Taiwan.
Footnote
Conflicts of Interest: All authors have completed the ICMJE uniform disclosure form (available at http://dx.doi.org/10.21037/qims-20-777). The authors have no conflicts of interest to declare.
Ethical Statement: All of the experimental procedures conformed to the guidelines of the National Institute of Health, and were approved by the Animal Care and Use Committee of Chi-Mei Medical Center (Affidavit of approval of Animal Use Protocol, Chi-Mei Medical Center, IACUC Approval No. 102120603) to minimize discomfort to the animals during the surgery and recovery periods.
Open Access Statement: This is an Open Access article distributed in accordance with the Creative Commons Attribution-NonCommercial-NoDerivs 4.0 International License (CC BY-NC-ND 4.0), which permits the non-commercial replication and distribution of the article with the strict proviso that no changes or edits are made and the original work is properly cited (including links to both the formal publication through the relevant DOI and the license). See: https://creativecommons.org/licenses/by-nc-nd/4.0/.
References
- Gupta AK. Monitoring the injured brain in the intensive care unit. J Postgrad Med 2002;48:218-25. [PubMed]
- Ganau M, Prisco L. Comment on "neuromonitoring in traumatic brain injury". Minerva Anestesiol 2013;79:310-1. [PubMed]
- Syrmos N, Ganau M, De Carlo A, Prisco L, Ganau L, Valadakis V, Grigoriou K, Iliadis C, Arvanitakis D. Dealing with the surgical and medical challenges of penetrating brain injuries. Case Rep Surg 2013;2013:209750 [Crossref] [PubMed]
- Ganau M, Lavinio A, Prisco L. Delirium and agitation in traumatic brain injury patients: an update on pathological hypotheses and treatment options. Minerva Anestesiol 2018;84:632-40. [PubMed]
- Meixensberger J, Jaeger M, Väth A, Dings J, Kunze E, Roosen K. Brain tissue oxygen guided treatment supplementing ICP/CPP therapy after traumatic brain injury. J Neurol Neurosurg Psychiatry 2003;74:760-4. [Crossref] [PubMed]
- Jaeger M, Soehle M, Schuhmann M, Winkler D, Meixensberger J. Correlation of continuously monitored regional cerebral blood flow and brain tissue oxygen. Acta Neurochirurgica 2005;147:51-6. [Crossref] [PubMed]
- Friess SH, Ralston J, Eucker SA, Helfaer MA, Smith C, Margulies SS. Neurocritical care monitoring correlates with neuropathology in a swine model of pediatric traumatic brain injury. Neurosurgery 2011;69:1139-47. [Crossref] [PubMed]
- Barazangi N, Hemphill JC III. Advanced cerebral monitoring in neurocritical care. Neurology India 2008;56:405-14. [Crossref] [PubMed]
- Ferrari M, Giannini I, Sideri G, Zanette E. Continuous non invasive monitoring of human brain by near infrared spectroscopy. Adv Exp Med Biol 1985;191:873-82. [Crossref] [PubMed]
- Wyatt JS, Cope M, Delpy DT, Wray S, Reynolds EO. Quantification of cerebral oxygenation and haemodynamics in sick newborn infants by near infrared spectrophotometry. Lancet 1986;2:1063-6. [Crossref] [PubMed]
- Hasan S, Chari A, Ganau M, Uff C. Defining New Research Questions and Protocols in the Field of Traumatic Brain Injury through Public Engagement: Preliminary Results and Review of the Literature. Emerg Med Int 2019;2019:9101235 [Crossref] [PubMed]
- Ganau M, Iqbal M, Ligarotti GKI, Syrmos N. Breakthrough in the assessment of cerebral perfusion and vascular permeability after brain trauma through the adoption of dynamic indocyanine green-enhanced near-infrared spectroscopy. Quant Imaging Med Surg 2020;10:2081-4. [Crossref] [PubMed]
- Kuo JR, Lin BS, Cheng CL, Chio CC. Hypoxic-state estimation of brain cells by using wireless near-infrared spectroscopy. IEEE J Biomed Health Inform 2014;18:167-73. [Crossref] [PubMed]
- Delpy DT, Cope M, van der Zee P, Arridge S, Wray S, Wyatt J. Estimation of optical pathlength through tissue from direct time of flight measurement. Phys Med Biol 1988;33:1433-42. [Crossref] [PubMed]
- Ferrari M, Cettolo V, Quaresima V. Light source-detector spacing of near-infrared-based tissue oximeters and the influence of skin blood flow. J Appl Physiol (1985) 2006;100:1426; author reply 1427.
- McIntosh TK, Vink R, Noble L, Yamakami I, Fernyak S, Soares H, Faden AL. Traumatic brain injury in the rat: characterization of a lateral fluid-percussion model. Neuroscience 1989;28:233-44. [Crossref] [PubMed]
- Werner C, Engelhard K. Pathophysiology of traumatic brain injury. Br J Anaesth 2007;99:4-9. [Crossref] [PubMed]
- Carlson BE, Arciero JC, Secomb TW. Theoretical model of blood flow autoregulation: roles of myogenic, shear-dependent, and metabolic responses. Am J Physiol Heart Circ Physiol 2008;295:H1572-9. [Crossref] [PubMed]
- Frank JI. Large hemispheric infarction, deterioration, and intracranial pressure. Neurology 1995;45:1286-90. [Crossref] [PubMed]
- Grubb RL Jr, Raichle ME, Phelps ME, Ratcheson RA. Effects of increased intracranial pressure on cerebral blood volume, blood flow, and oxygen utilization in monkeys. J Neurosurg 1975;43:385-98. [Crossref] [PubMed]
- Kobari M, Gotoh F, Tomita M, Tanahashi N, Shinohara T, Terayama Y, Mihara B. Bilateral hemispheric reduction of cerebral blood volume and blood flow immediately after experimental cerebral hemorrhage in cats. Stroke 1988;19:991-6. [Crossref] [PubMed]
- Johnston AJ, Steiner LA, Coles JP, Chatfield DA, Fryer TD, Smielewski P, Hutchinson PJ, O'Connell MT, Al-Rawi PG, Aigbirihio FI, Clark JC, Pickard JD, Gupta AK, Menon DK. Effect of cerebral perfusion pressure augmentation on regional oxygenation and metabolism after head injury. Crit Care Med 2005;33:189-95; discussion 255-7. [Crossref] [PubMed]
- Marchal G, Young AR, Baron JC. Early postischemic hyperperfusion: pathophysiologic insights from positron emission tomography. J Cereb Blood Flow Metab 1999;19:467-82. [Crossref] [PubMed]
- Stocchetti N, Chieregato A, De Marchi M, Croci M, Benti R, Grimoldi N. High cerebral perfusion pressure improves low values of local brain tissue O2 tension (PtiO2) in focal lesions. Acta Neurochir Suppl 1998;71:162-5. [Crossref] [PubMed]
- Forcione M, Yakoub KM, Chiarelli AM, Perpetuini D, Merla A, Sun R, Sawosz P, Belli A, Davies DJ. Dynamic contrast-enhanced near-infrared spectroscopy using indocyanine green on moderate and severe traumatic brain injury: a prospective observational study. Quant Imaging Med Surg 2020;10:2085-97. [Crossref] [PubMed]
- Forcione M, Chiarelli AM, Davies DJ, Perpetuini D, Sawosz P, Merla A, Belli A. Cerebral perfusion and blood-brain barrier assessment in brain trauma using contrast-enhanced near-infrared spectroscopy with indocyanine green: A review. J Cereb Blood Flow Metab 2020;40:1586-98. [Crossref] [PubMed]
- Shepelytskyi Y, Hane FT, Grynko V, Li T, Hassan A, Albert MS. Hyperpolarized 129Xe Time-of-Flight MR Imaging of Perfusion and Brain Function. Diagnostics (Basel) 2020;10:630. [Crossref] [PubMed]
- Adjedj J, Picard F, Vanhaverbeke M, De Bruyne B, Cariou A, Wu M, Janssens S, Varenne O. A novel approach to assess cerebral and coronary perfusion after cardiac arrest. Intensive Care Med Exp 2018;6:39. [Crossref] [PubMed]
- Fantini S, Sassaroli A, Tgavalekos KT, Kornbluth J. Cerebral blood flow and autoregulation: current measurement techniques and prospects for noninvasive optical methods. Neurophotonics 2016;3:031411 [Crossref] [PubMed]