Assessment of oxygen saturation in retinal vessels of normal subjects and diabetic patients with and without retinopathy using Flow Oximetry System
Introduction
Diabetes mellitus (DM) is a metabolic disease that causes considerable worldwide morbidity and mortality. In 2010, researchers estimated that 285 million people worldwide had diabetes, and that these numbers will probably increase by 54% by 2030 (1). In the United States, about 10.9 million persons were diagnosed with diabetes in 2010 (2). Among the many complications of DM, the micro-vascular morbidities can be quite severe and can affect multiple organ systems resulting in complications such as retinopathy, nephropathy, and neuropathy, among others. Diabetic retinopathy (DR) is ranked as the leading cause of blindness and visual disability in the middle-aged/working American population (3), and these complications can lead to a reduction in life expectancy and can have a considerable impact on quality- and disability-adjusted life years indices as well as the cost of health care for affected patients (4). Therefore, it is essential to understand the progression of the disease with an aim to possibly prevent or delay the development of its complications.
Though the pathogenesis of DR is not fully understood, DR is believed to be associated with changes in oxygen saturation (StO2) in retinal vessels (5), and its development has been linked to changes in partial pressure of oxygen (pO2) in the retina (6). In addition, hypoxia is one of the main factors involved in the pathogenesis of DR, and eventually neovascularization (7). We believe that showing the changes in retinal oxygenation and metabolism in patients with no or early retinopathy will give clinicians a better and earlier insight on diabetic progression. However, since early microvascular changes may not be easily detected on clinical examination compared to late changes, at which point they are usually irreversible, changes in the hemoglobin StO2 in retinal arteries and veins may indicate very early microangiopathy, which could help clinicians detect vascular compromise in diabetic patients long before retinopathy is seen clinically. Recently several devices have appeared, both in laboratory and clinical settings, capable of monitoring either StO2 (8-11) in the retinal arterioles and venules or flow velocity in the superficial retina capillary network (12,13).
The Flow Oximetry System (FOS) (Figure 1) is a novel non-invasive system developed by our group to measure retinal StO2 and retinal blood flow. The FOS system is capable of calculating StO2 in the retina by acquiring spectroscopic sensitive images of the retinal vessels, making it the first device to simultaneously measure retinal oxygenation and blood flow. The device used in this study is a modified dual-length version of earlier prototypes that has been reported elsewhere (14). The results obtained with the FOS were co-registered with optical coherence tomography (OCT) thickness map obtained with a commercial system (Spectralis HRA+OCTTM, Heidelberg Engineering Inc., Vista, CA, USA). OCT is a non-invasive, non-contact imaging technique that produces high resolution, cross-sectional images of human tissue. Recent work has suggested that change in retinal thickness, and more importantly the volume, can be used to assess the rate of change of disease, or progression and regression. In patients with macular edema followed by the vascular damage, the earliest change detected was a collection of excessive fluid in the Muller cells. After the loss of Muller cells, the fluid accumulated in the outer plexiform layer of Henle. The photoreceptor layer may be damaged due to this increased intraretinal pressure. Thus, OCT thickness and volume may serve as potential useful indicators of early change the patients with diabetes.)
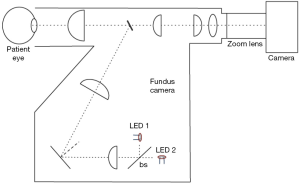
Methods
Flow Oximetry System (FOS)
The new FOS uses a simple two-wavelength algorithm to ascertain StO2 levels in the retina. This method was developed by other groups (15-18) and was adapted to our instrumentation. The flow assessment is conducted with a red-blood-cells-tracking technique previously presented by our group (19).
The combined flow and oximetry system consists of a modified Fundus Camera (Zeiss FF3, Jena, Germany), where both the original light sources (white light lamp for focusing and a flash light for image acquisition) were replaced with two light engines (Enfis, Swansea, UK, LED1 and LED 2 in Figure 1). The light engines were centered at 520 and 630 nm (15 nm FWHM). It is to note that Bosschaart et al. have shown 522 nm to be an isosbestic wavelength in human blood (20). A 30:70 beam-splitter was used to maximize the throughput of the green light source so to optimize image quality. This was necessary due to the low backscattered remission from the retina in that wavelength range. A color camera (24 bit, Prosilica Genie, Billerica, Massachusetts, 1,024 pixels × 1,024 pixels, chip size 3 mm × 3 mm) was combined with a zoom lens with focal length of 150-450 mm, f/5.6-f/3.2, (Computar, Commack, New York) and connected to the fundus system through its imaging port. A custom black epoxy attachment was constructed to align the camera to the system as well as obtain a solid connection between camera and fundus system. The camera was capable of 60 frames per second acquisition.
Image acquisition, was controlled through a custom made software, (Matlab®, MathWorks, Natick, Massachusetts). After the software was started, the camera entered focus mode where only the green light engine was active. In this mode, the engine was pulsated at 60 Hz and controlled by the camera; pulses were 3 µs in duration and camera gain was set to maximum. A short delay 0.5 µs between camera acquisition and pulse start was added programmatically to avoid any issues with the light source ramp up time. The short pulse setting was used to focus the fundus system and allow the operator to locate a region of interest within the patient retina. At the same time by using these settings, the subject compliance and comfort were maximized. When the operator considered all imaging parameters to be satisfactory, image acquisition was triggered through either a button in the general user interface or with a foot pedal connected to a data acquisition card (National Instruments, Austin, Texas) controlled by the program. The acquisition phase lasted a total of 4 seconds equal to 240 frames. During acquisition both the red and green light engine were active at 60 Hz. The pulse duration was increased to 6 µs so to have more energy deposition and clearer images. Even with this larger pulse the energy level of the combined light engines was well below the retina threshold of damage. Once the acquisition time was expired, the 240 frames were saved in an uncompressed Audio Video Interleave (AVI) format.
The color images produced by the camera were processed into their basic Bayer components; Red, Green, and Blue images. The 240 frames stack of Green images was used for the flow assessment, while both Red and Green stacks were used for the oximetry measurements. Minor cross talk between the images was noticed. The stacks of images were also registered with an algorithm presented elsewhere (19). Frames with large movement artifacts were discarded, both techniques can utilize as little as 20 frames so only a stable segment (small to no-motion present) of the full stack was ultimately used. When measuring oxygen within the retinal capillaries, frames for the Red and Green stack were averaged producing an average R and G image. These two images were then processed using the aforementioned algorithm based on calculation of the vessel optical density, where reflectance values on the vessels where normalization by reflectance values of nearby background.
Images obtained with an OCT system were co-registered with the FOS maps. A simple algorithm was devised for this purpose. Three characteristics points were selected in both the FOS image and the OCT enface image (typically vessel bifurcations were used), once the coordinate of the location were known, the OCT enface and thickness map were padded through interpolation to the same size as the FOS image. Since the thickness maps were generated with approximately 20 OCT scans, this interpolation did not drastically reduce the OCT thickness resolution. An example of this process is shown in Figure 2.
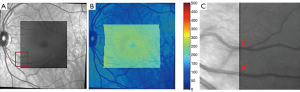
In vitro calibration and validation of two-wavelength system was conducted utilizing an eye phantom (21) combined with a 100 µm diameter microfluidic channel (Translume, Ann Arbor, MI), an epoxy phantom background mimicking various layer of the retina (RPE, Choroid, and Sclera) and a syringe pump (Harvard Apparatus, Holliston, MA). The choice of vessel size for in vitro testing was determined by our system limitations; while the velocity assessment works best at low vessel size the opposite is true for StO2 measurements (22).
While our previously reported (14) multi-wavelength system allowed for direct StO2 extrapolation through minimization of the intensity values of light backscattered from capillaries to the curves of oxygenated and deoxygenated hemoglobin, the two wavelengths system uses a different principle based on monitoring of vessel optical density. Hence the validation approach was based on measuring the optical densities [OD =log (Ibackground/Ivessel)] for the two wavelength of interest (OD520 for 520 nm and OD630 for 630 nm) on vessels of known diameter (100 µm) that were filled with known concentration of human blood from a local blood bank. StO2 of the blood was modified through the addition of sodium hydrosulfite (Sigma, St. Louis, Missouri). Values between 50% and 100% StO2 were created, as they were considered physiologically relevant. Before being imaged with the FOS, the same samples were measured with a bench-top oxygen sensor (Ocean Optics, Dundee, FL).
After FOS image acquisition, the OD ratio ODR = OD630/OD520 was calculated. Values of ODR measured with the FOS were linearly proportional to the ones measured with the spectrophotometer and could be related to the true values of StO2. Each measurement was repeated three times.
The results of the velocity calibration have been presented elsewhere (19), here we simply present an example of our in vitro results where the syringe pump flow rate is increased to three different levels. As a consequence, the velocity within the capillary increases.
Subjects and characterization
Our study was designed as a prospective case-series study to assess retinal StO2 in normal subjects, patients with DM without DR, and diabetic patients with non-proliferative diabetic retinopathy (NPDR). Our research adhered to the tenets of the Declaration of Helsinki (as revised in Edinburgh 2000) and was approved by the Johns Hopkins institutional review board. Patients were enrolled after giving their informed consent. Both type 1 and type 2 diabetics were included and duration of diabetes was noted. Subjects were required to have ocular media sufficiently clear to allow good quality ocular imaging. Exclusion criteria included subjects with corneal opacities, cataracts or dense vitreous hemorrhage, and patients with severe non-proliferative and proliferative DR. Current smokers and subjects with history of vitreo-retinal disease or surgery such as retinal detachment, epiretinal membrane, or vitreous hemorrhage were excluded. Other exclusion criteria included patients with other retinal or macular diseases other than that caused by diabetes and those with medical conditions that could interfere with the subject’s ability to comply with study procedure such as inability to maintain steady head or eye positioning, as in patients with ataxia or nystagmus. Pupils were dilated prior to the image acquisition by standard dilation procedures using proparacaine hydrochloride 0.5%, tropicamide 1%, and phenylephrine hydrochloride 2.5%.
After dilation, a 4-second video was acquired. A total of 240 color frames (in 4 seconds) were acquired from each study eye. Analysis software was developed and used to calculate StO2. Because the optical properties of the layers underlying the retinal blood vessels vary from one patient to another, a calibration step was required at the beginning of the image analysis for every patient. The software provided a manual mode for tracing the segments of vessels to be analyzed and the calculated StO2 levels of the vessels were then overlaid on the gray scale image of the retina with false colors representation.
The FOS computerized assessment software was used to determine StO2 levels in arteries and veins located in two main areas of the retina. The first area was the central 6 mm of the retina, excluding the foveal avascular zone (central 1 mm), and the second area was between 1 to 2 mm from the margin of the optic disc. While in the first area, flow, oxygenation, and retinal thickness could be measured, the vessels surrounding the optics discs were too large for our flowmetry technique and retinal thickness was not measured, hence only oxygenation was considered. The image pixel size, which is calculated during the calibration portion of the study, was used to measure distances across the fundus image. The images were further sub-divided into four quadrants: superior, inferior, temporal, and nasal.
For the first study a total of 77 subjects (77 eyes) were included in the final analyses (31 normal subjects, 25 DM no DR patients, and 21 NPDR patients). Table 1 shows the demographics and baseline characteristics of the study participants in different study groups. Measurements of the StO2 and flow velocity were conducted. The measurements were made through tracing all vasculature in the central 6 mm of the retina, excluding the foveal avascular zone (central 1 mm). Arteries and veins were identified and traced. The average StO2, velocities, and retina thickness, along single vessel and across multiple vessels and two main zones (1 and 2 mm diameter from the macula) was calculated.
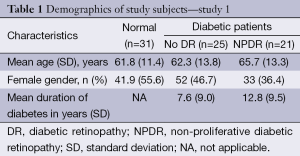
Full table
For the second study a total of 44 subjects (44 eyes) were included in the final analyses (18 normal subjects, 15 DM no DR patients, and 11 NPDR patients). Table 2 shows the demographics and baseline characteristics of the study participants in different study groups. Age of participants ranged from 30 to 83 years, with the mean [± standard deviation (SD)] of 61 (±12.5) years. The mean duration of diabetes in patients without retinopathy was 5.2 (±3.2) years and was 12.8 (±11.6) years in patients with retinopathy. 61.1% of our patients were Caucasian, 22.2% were African Americans, 11.1% Asians, and 5.6% belonged to other races. In this phase of the study arteries and veins from either the superior and/or inferior quadrants were selected. Vessels within the temporal and nasal quadrants were excluded because of their small size. Measurements were taken from one major artery and one major vein in each quadrant. Only one eye per patient and only vessels clearly identified by an experienced ophthalmologist as arteries and veins were included in the final analysis. Eyes in which at least one artery and one vein in either quadrant could not be measured were excluded from the analysis. Vessel tracing was done manually and calculation of the StO2 was automatically done using the computerized software. Patients in whom the duration of diabetes was unknown were eliminated from the final analyses.
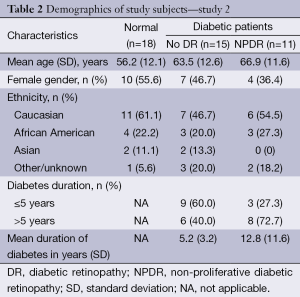
Full table
Data was presented as mean and standard deviation (SD). Comparisons of means were done using variance analysis (ANOVA). Pairwise comparisons of the three groups of the study (normal vs. DM no DR, normal vs. NPDR, and DM no DR vs. NPDR) were performed using Bonferroni post-hoc analysis. All analyses were done with STATA statistical software, version 11 (STATA Corp, Texas).
Results
In vitro results
Various values of StO2 of human blood were used during this phase of testing and the results obtained from the FOS system were compared to the one obtained with the oxygen sensor. ODR at the chosen wavelengths decreases linearly with increased StO2; ODR data was fitted with a first order polynomial to minimize some of the experimental error. The results of this approach are shown in the insert of Figure 3. The corrected values of ODR were ultimately used to calculate StO2. Results are shown in Figure 3.
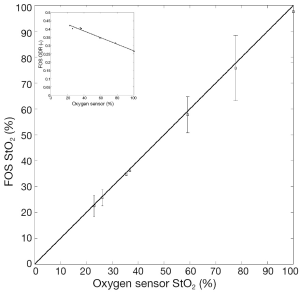
Figure 4 shows typical trace history map for increasing pump flow rate. The trace histories correspond to values of flow rate between 0.01 and 0.05 mL/h.
In vivo results
Figures 5 and 6 show results obtained in the first region under investigation (macular area). The region in the insert of Figure 5 were averaged and separated into two data sets. Our analysis of flow and StO2 values did not reveal any statistical significance when comparing study groups. Figure 7 shows the average retinal thickness in the macular region.
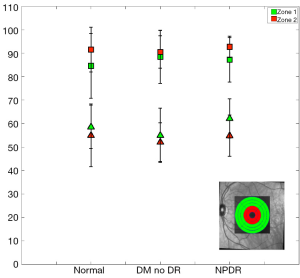
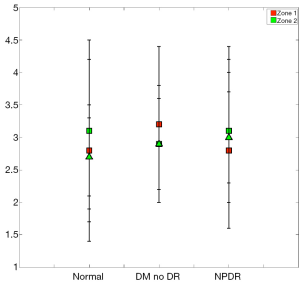
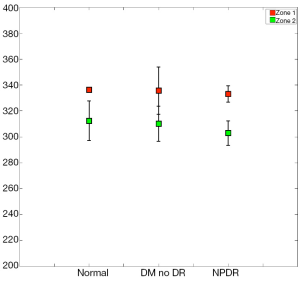
When imaging areas in the proximity of the optic disc (Figure 8), some statistical differences arose. The mean StO2 (±SD) in retinal arteries was 96.9%±3.8% (median; 98.9%), in normal subjects, 97.2%±3.6% (median; 98.9%) in patients with DM without DR, and 98.4%±2.0% (median; 99.1%) in patients with NPDR (Table 3). The mean venous StO2 was 57.5%±6.8% (median; 57.7%) in normal subjects, 57.4%±7.3% (median; 58.1%) in patients with DM without DR, and 51.8%±6.8% (median; 51.4%) in patients with NPDR (Table 3). Among all patients, the minimum measured arterial StO2 was 90.1% and the maximum was 100%, while the minimum measured venous StO2 was 43.8% and the maximum was 66.8%.

Full table
While the arterial StO2 tended to be highest in patients with NPDR when compared to the other groups, the venous StO2 tended to be lowest in this same group of patients (Table 3). Nevertheless, no statistically significant difference was found when the mean arterial and mean venous StO2 were compared across the three groups using the one-way ANOVA test (Table 4). Comparison of the mean arterio-venous (A-V) difference across the three groups, however, was found to be statistically different (P=0.015) (Figure 9). Pairwise comparisons of the mean A-V difference among the three groups, adjusted for multiple comparisons, using Bonferroni’s post-hoc analysis (Tables 3,4) demonstrated statistically significant differences between the normal subjects and the NPDR group (P=0.02) and between diabetic patients without DR and patients with NPDR (P=0.042). However, no statistically significant difference was found when we compared the A-V difference for normal subjects and diabetic patients without DR (2).
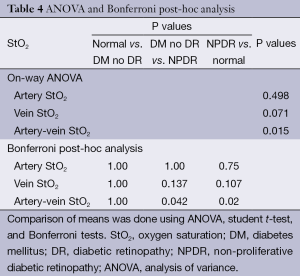
Full table
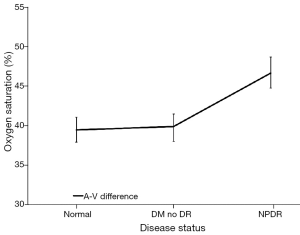
Though we did not compare the mean StO2 across the different quadrants, there were no major differences in the measured StO2 values between the superior or inferior quadrants.
The retinal vascular system is an environment where a constant balance of oxygen pressure and StO2 is required for normal functioning of the eye (23). Therefore, changes in StO2 may indicate onset of hypoxia and microvascular retinal changes that may aid in clinical diagnosis of early diabetic eye changes. In our study, we found that, compared to individual changes in StO2 of retinal veins and arteries, calculation of the A-V differences might be a better predictor of tissue oxygenation. Such finding can be explained by Fick’s principle, which states that the consumption of oxygen in a tissue is proportional to the rate of blood flow through that tissue times the difference in StO2 between the arterial and venous circulations in the same tissue (24). It is important to note that studies exploring the regional oxygen differences in the retina found that vessels further away from the macula demonstrated lower arterial and venous StO2 compared to vessels closer to the macula, independent of vessel diameter (23). Skov Jensen et al. have also suggested that peripheral and macular retinal blood vessels show differences in their ability to autoregulate their metabolic needs; this was true in healthy individuals as well as in patients with peripheral or macular DR (25). Therefore, depending on the location of measurement of StO2 in the retina, the results can differ widely. To avoid such location-dependent variation of StO2, the measurements in our study were taken from vessels in standard location in the peri-papillary region.
Our results show that diabetics with NPDR were more likely to have a higher arterial but lower venous StO2 with statistically significant higher A-V difference when compared to normal subjects and to diabetic patients without retinopathy. This is contrary to the results reported by Hammer et al. 2009 where patients with mild NPDR had lower A-V difference when compared to normal subjects (9). Hardarson and Stefánsson 2012 reported a higher venous StO2 in patients with DR. However in their study, they have stratified their sample to patients with background retinopathy, patients with macular edema, and patient with pre-PDR/PDR. Therefore, it is unlikely that our results can be directly compared as it is unknown where patients with mild NPDR would fit in such classification (5).
The increase in A-V difference in the NPDR group compared to the other groups could be due to an increase in oxygen consumption by the retina, a likely compensatory mechanism for the onset of hypoxia in some areas of the retina. Physiologically, the decrease in oxygen supply to a tissue usually sets off a cascade of adaptive mechanisms designed to maintain cellular activity at the lowest acceptable level to ensure survival of the affected tissue. Continued or worsening hypoxia leads to a failure of this compensatory mechanism, leading to cellular dysfunction and possibly irreversible cell damage (26). In mildly hypoxic but still viable retina as could be the case in mild NPDR, there may be increased extraction of oxygen the retina, which leads to a lower venous StO2 and a higher A-V difference as evidenced in our study. As hypoxia worsens with further progression of the retinopathy, the number of viable areas in the retina will eventually decrease; at that stage, we may begin to see a decrease in the ability of the tissue to use oxygen, which may explain the higher venous StO2 and lower A-V difference observed in the other studies. Calculating the A-V difference in the same vessels at the same location and monitoring such A-V gradient over time may give a more accurate representation of disease progression.
Tiedeman et al. have found that the decrease in venous StO2 in diabetic patients to be highly correlated with the glycemic state of the patient, as well as in patients who had a longer duration of diabetes (24), which is consistent with what we have observed in our study (Table 1). The authors have postulated that venous StO2 in the retina depends on an auto-regulatory response in retinal tissues driven by oxygen demand and patients who were not able to auto-regulate adequately to an increasing oxygen demand, tended to extract more oxygen from the blood and subsequently had lower venous StO2 (24). Patients with longer duration of diabetes are more prone to have uncontrolled hyperglycemia; therefore, it is plausible that the longer the duration of diabetes the higher the probability of retinopathy and hence, the increased probability of lower venous StO2.
Increased oxygen delivery to the retinal tissue can also be explained in the absence of retinal hypoxia. Diabetic patients usually have higher metabolic demand, which is further complicated by the increase in glycosylated hemoglobin (HbA1c), which has higher affinity to oxygen. However, the insulin deficiency/resistance in those patients leads to increased levels of 2,3-bisphosphoglycerate (2,3-BPG) in the red blood cells (27). 2,3-BPG shifts the oxygen dissociation curve to the right, increasing oxygen delivery to the retinal tissues. The net result of increased affinity of HbA1c to oxygen and the increase in 2,3-BPG is complex and it is possible that early in the disease, the increased oxygen delivery to retinal tissues, as observed in our study, is derived by the dominance of the increased levels of 2,3-BPG.
Finally, the increase in A-V difference in the NPDR group may not indicate increased oxygen delivery to tissues. According to Fick’s principle, oxygen consumption is the result of retinal blood flow and A-V difference. Therefore, the increase in oxygen extraction as indicated by the increased A-V difference in NPDR group can be negated by decreased retinal blood flow.
Our initial attempt to measure the StO2 in the macular region has not showed any statistically significant differences or particular trends in all study groups either in StO2 of blood velocity. This was true, whether the measured vessel is an artery, a vein, or representing an A-V differential. We believe this was the result of several factors. First, it was very difficult, even for experienced ophthalmologist, to differentiate 3rd and 4th order arteries from veins giving the very small caliber of the measured vessels. Second, in many instances, there were not enough vessels that could be confidently measured in the central 6 mm zone, especially in patients with NPDR, which resulted in exclusion of large number of data points with subsequent significant reduction of our sample size and hence, our statistical power. Finally, with very small vessels, the SDs of the averages along any measured vessel, whether it is a potential artery or vein, but especially with proposed arteries were too high.
Conclusions
We have described a normative range of arterial and venous StO2 as obtained using the FOS and have shown that while arterial StO2 tended to increase mildly in patients who have NPDR, venous StO2 tended to decrease in this same group of patients when compared to the other groups, with significant increase in A-V difference. Our findings suggest increased oxygen extraction in eyes with early DR, which can be due to increased oxygen consumption by retinal tissue or secondary to a compensatory mechanism in response to reduction of blood flow in areas of retinal hypoxia. It is possible to explain the increase in oxygen extraction by right shift of the oxygen dissociation curve secondary to increased 2, 3-DPG levels or possibly a combination of all previous mechanisms. Our study also suggests that A-V difference could be a more accurate predictor of tissue oxygenation compared to measuring either retinal arterial or venous StO2 alone.
Additional studies with a larger sample size, blood flow measurements, and inclusion of patients with more advanced/proliferative DR are indicated to confirm our findings and to further elucidate the utility of the FOS in clinical settings.
Acknowledgements
We express our deep appreciation to the Wilmer Biostatistics Department, which provided statistical support to the data analyses of this study. The study is supported in part by a grant from the National Eye Institute, National Institutes of Health (RO1 EY017577 for QDN).
Disclosure: At the time of submission, the submitting authors have not published or submitted the index manuscript elsewhere. All authors of this manuscript do not have relationships with companies that may have a financial interest in the information contained in the manuscript
References
- Shaw JE, Sicree RA, Zimmet PZ. Global estimates of the prevalence of diabetes for 2010 and 2030. Diabetes Res Clin Pract 2010;87:4-14. [PubMed]
- U.S. department of health and human services cfdcap. National diabetes fact sheet: National estimates and general information on diabetes and prediabetes in the united states, 2011. Atlanta, GA2011 [cited 2011]; Available online: http://www.cdc.gov/diabetes/pubs/pdf/ndfs_2011.pdf
- WHO. Global strategy on diet, physical activity and health. 2004; Available online: http://www.who.int/dietphysicalactivity/publications/facts/diabetes/en/
- Girach A, Manner D, Porta M. Diabetic microvascular complications: can patients at risk be identified? A review. Int J Clin Pract 2006;60:1471-83. [PubMed]
- Hardarson SH, Stefánsson E. Retinal oxygen saturation is altered in diabetic retinopathy. Br J Ophthalmol 2012;96:560-3. [PubMed]
- Hammer M, Riemer T, Vilser W, Gehlert S, Schweitzer D. A new imaging technique for retinal vessel oximetry: principles and first clinical results in patients with retinal arterial occlusion and diabetic retinopathy. Proc SPIE 7163 Ophthalmic Technologies 2009;XIX:71630P. [PubMed]
- Engerman RL. Pathogenesis of diabetic retinopathy. Diabetes 1989;38:1203-6. [PubMed]
- Mordant DJ, Al-Abboud I, Muyo G, Gorman A, Harvey AR, McNaught AI. Oxygen saturation measurements of the retinal vasculature in treated asymmetrical primary open-angle glaucoma using hyperspectral imaging. Eye (Lond) 2014;28:1190-200. [PubMed]
- Hammer M, Vilser W, Riemer T, Mandecka A, Schweitzer D, Kühn U, Dawczynski J, Liemt F, Strobel J. Diabetic patients with retinopathy show increased retinal venous oxygen saturation. Graefes Arch Clin Exp Ophthalmol 2009;247:1025-30. [PubMed]
- Schweitzer D, Thamm E, Hammer M, Kraft J. A new method for the measurement of oxygen saturation at the human ocular fundus. Int Ophthalmol 2001;23:347-53. [PubMed]
- Beach J, Ning J, Khoobehi B. Oxygen saturation in optic nerve head structures by hyperspectral image analysis. Curr Eye Res 2007;32:161-70. [PubMed]
- Grunwald JE, Riva CE, Baine J, Brucker AJ. Total retinal volumetric blood flow rate in diabetic patients with poor glycemic control. Invest Ophthalmol Vis Sci 1992;33:356-63. [PubMed]
- Grinvald A, Bonhoeffer T, Vanzetta I, Pollack A, Aloni E, Ofri R, Nelson D. High-resolution functional optical imaging: from the neocortex to the eye. Ophthalmol Clin North Am 2004;17:53-67. [PubMed]
- Lemaillet P, Duncan DD, Lompado A, Ibrahim M, Nguyen QD, Ramella-Roman JC. Retinal Spectral Imaging and Blood Flow Measurement. J Innov Opt Heal Sci 2010;3:255-65.
- Hardarson SH, Harris A, Karlsson RA, Halldorsson GH, Kagemann L, Rechtman E, Zoega GM, Eysteinsson T, Benediktsson JA, Thorsteinsson A, Jensen PK, Beach J, Stefánsson E. Automatic retinal oximetry. Invest Ophthalmol Vis Sci 2006;47:5011-6. [PubMed]
- Hardarson SH, Basit S, Jonsdottir TE, Eysteinsson T, Halldorsson GH, Karlsson RA, Beach JM, Benediktsson JA, Stefansson E. Oxygen saturation in human retinal vessels is higher in dark than in light. Invest Ophthalmol Vis Sci 2009;50:2308-11. [PubMed]
- Hammer M, Vilser W, Riemer T, Liemt F, Jentsch S, Dawczynski J, Schweitzer D. Retinal venous oxygen saturation increases by flicker light stimulation. Invest Ophthalmol Vis Sci 2011;52:274-7. [PubMed]
- Beach JM, Schwenzer KJ, Srinivas S, Kim D, Tiedeman JS. Oximetry of retinal vessels by dual-wavelength imaging: calibration and influence of pigmentation. J Appl Physiol (1985) 1999;86:748-58. [PubMed]
- Duncan DD, Lemaillet P, Ibrahim M, Nguyen QD, Hiller M, Ramella-Roman J. Absolute blood velocity measured with a modified fundus camera. J Biomed Opt 2010;15:056014. [PubMed]
- Bosschaart N, Edelman GJ, Aalders MC, van Leeuwen TG, Faber DJ. A literature review and novel theoretical approach on the optical properties of whole blood. Lasers Med Sci 2014;29:453-79. [PubMed]
- Lemaillet P, Ramella-Roman JC. Dynamic eye phantom for retinal oximetry measurements. J Biomed Opt 2009;14:064008. [PubMed]
- Geirsdottir A, Palsson O, Hardarson SH, Olafsdottir OB, Kristjansdottir JV, Stefánsson E. Retinal vessel oxygen saturation in healthy individuals. Invest Ophthalmol Vis Sci 2012;53:5433-42. [PubMed]
- Heitmar R, Safeen S. Regional differences in oxygen saturation in retinal arterioles and venules. Graefes Arch Clin Exp Ophthalmol 2012;250:1429-34. [PubMed]
- Tiedeman JS, Kirk SE, Srinivas S, Beach JM. Retinal oxygen consumption during hyperglycemia in patients with diabetes without retinopathy. Ophthalmology 1998;105:31-6. [PubMed]
- Skov Jensen P, Jeppesen P, Bek T. Differential diameter responses in macular and peripheral retinal arterioles may contribute to the regional distribution of diabetic retinopathy lesions. Graefes Arch Clin Exp Ophthalmol 2011;249:407-12. [PubMed]
- Pierson DJ. Pathophysiology and clinical effects of chronic hypoxia. Respir Care 2000;45:39-51; discussion 51-3. [PubMed]
- Ditzel J. The problems of tissue oxygenation in diabetes mellitus. III. The “three-in-one concept” for the development of diabetic microangiopathy and a rational approach to its prophylaxis. Acta Med Scand Suppl 1975;578:69-83. [PubMed]