Technical challenges in 3 T magnetic resonance spectroscopic imaging of the prostate—A single-institution experience
Introduction
Magnetic resonance imaging (MRI) has shown a significant impact on clinical decision making in prostate cancer management (1). In recent years, the multiparametric MRI, which includes any combination of dynamic contrast-enhanced (DCE) MRI, diffusion-weighted MRI (DWI), and magnetic resonance spectroscopic imaging (MRSI), has been advocated to better localize and characterize prostate cancer and to improve the specificity of conventional MRI in detecting prostate cancer, although each technique has its own limitations and all of them are continuously being evolving (1-5). The DWI and DCE-MRI have been relatively well accepted and cautiously incorporated into the routine clinical practice of prostate MRI in many imaging centers. In contrast, MRSI of the prostate is still restricted to only a few academic institutions, despite the fact that MRSI is the only imaging technique that can evaluate the status of tissue metabolism that is specific to prostate tissue (6-11). A major hindrance to the clinical acceptance of prostate MRSI is the technical challenge in acquiring high-quality MRSI data.
MRSI at 1.5 T is commercially available through major vendors, and its use has been relatively well established in clinical practice at major academic institutions where a team of experts are available. Increasing the field strength from 1.5 to 3 T can theoretically provide proportionately higher signal-to-noise ratio (SNR) and improve spectral separation between prostatic metabolite peaks (Figure 1) and, thus, help radiologists more accurately interpret the images. However, compared with 1.5 T MRSI, 3 T MRSI requires some changes in acquisition technique and scan parameters to achieve expected high spectral quality. First, the echo time (TE) must be decreased from approximately 130 ms to approximately 85 ms in order to avoid a potential spectral distortion from the strongly coupled citrate spin system. Additionally, special attention needs to be paid to the increased susceptibility-induced magnetic field inhomogeneity and strong radiofrequency (RF) dielectric effects at 3 T. Without optimization of the increased field inhomogeneity and RF dielectric effect, the spectral peak broadening and residual signal from the periprostatic fat tissue may render the overall spectra non-diagnostic (Figures 1,2).
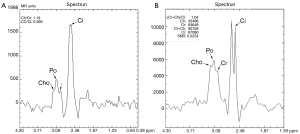
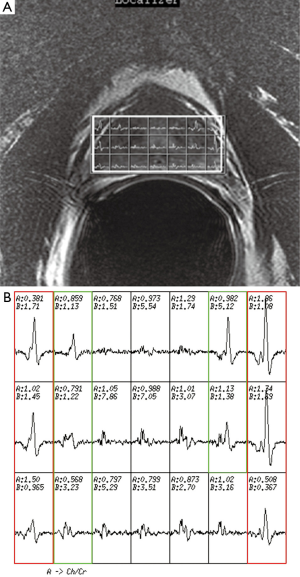
In this technical note, we describe our preliminary experience of a prototype 3 T MRSI (GE Health Care: GE, Milwaukee, WI) of the prostate in patients and how to manage some of the technical challenges to achieve optimal spectral quality.
Technical note
At our institution, the 3 T prostate MRSI is performed with an endorectal coil (e-coil) (MEDRAD, Pittsburg, PA, USA) to maximize the SNR. Proper placement of the e-coil is essential: care should be taken to keep the e-coil as straight as possible toward the midline of the body of patient (or prostate) to ensure that the sensitive volume of the coil is congruent with the prostate within approximately ±12 degrees from the midline. To minimize the magnetic field inhomogeneity introduced by the prostate-air interface between the prostate and rectum and endorectal coil balloon, the coil is filled with about 50-100 mL of perfluorocarbon complex (PFC) (3 M, St. Paul, MN, USA) (12). The magnetic susceptibility of PFC closely matches that of the prostatic tissues without generating any detectable MR signal (13). The proper positioning of the e-coil is visually confirmed by the three plane localizers (Figure 3).
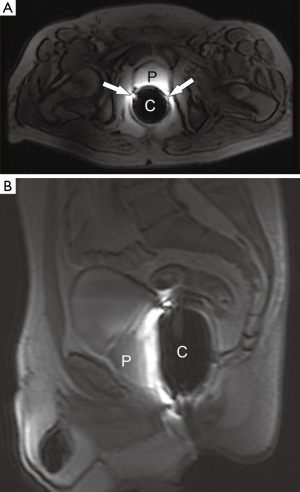
Once the position of the e-coil is confirmed, we acquire high-resolution (3 mm slice thickness without gap) T2-weighted images (T2WI) in three orthogonal planes before acquiring MRSI data. The MRSI acquisition parameters we typically use are repetition time (TR) =1,300 ms; TE =85 ms; number of excitations (NEX) =1; acquisition matrix =12×8×8; field of view =110×55×55 mm3; and a scan time of approximately 17 min.
The first step in prescribing an MRSI is to place a rectangular box of spectral volume of interest (VOI) on an axial T2WI on the largest cross-sectional area of the prostate. The size of the box is adjusted to maximize the coverage of the prostate with minimal inclusion of the periprostatic fat. A sagittal T2WI is used to further adjust the dimension of the VOI box along the superior-inferior direction to cover the prostate from the base to the apex, excluding the seminal vesicles in order to avoid spectral contamination from the seminal fluids (Figure 4). To further maximize the exclusion of the periprostatic fat, six very selective saturation (VSS) bands are placed (four over the axial image and two over the sagittal image) at the corners of the VOI to best conform the spectroscopic volume to the actual shape of the prostate (Figure 4).
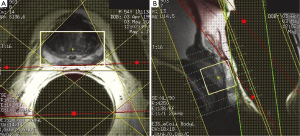
Once an appropriate VOI and the six VSS bands are placed, we perform an automatic prescan procedure that includes calibrating the transmit gains (TG) and receive gains, optimizing the magnetic field shimming, and calibrating the center transmit frequency. Optimum shimming of the magnetic field is key to the successful MRSI data acquisition. The quality of shimming can be checked by observing either the decay rate of the water free-induction decay signal or the shape of the water spectral peak in pure absorption mode after Fourier transformation. Alternatively, we can also display the water spectral peak in the magnitude mode and observe the full width at half maximum of the peak (in Hz). When the linewidth is suboptimal (e.g., >15 Hz), the settings for the three shimming gradients (along x, y, and z directions) can be adjusted manually to allow the free-induction decay signal to oscillate as far out as possible or to match the spectral shape in the pure absorption mode with that of the magnitude mode as closely as possible (Figure 5).
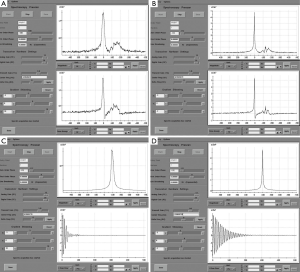
Another setting that we find requires careful tuning at 3 T is the TG, which is used to control the RF power output. An incorrect TG setting will result in an inaccurate flip angle of the RF pulses, which can lead to incomplete fat suppression and compromised spectral quality. Increased dielectric effects and B1 field shading at 3 T can make this effect particularly pronounced, especially in large patients.
To mitigate the sensitivity of the RF inhomogeneity at 3 T, the prototype software we use has “voxelated TG” as a default option. Voxelated TG only uses the signals from a small region of interest in the prostate, which is sensitive to the e-coil, for the purpose of calibrating the TG. Compared with using the signal from the built-in body coil at 1.5 T, voxelated TG is less sensitive to B1-inhomogeneity. However, we found that, in certain patients, the automatic prescan using the voxelated TG failed to complete the procedure. The root cause of this failure that we identified was the insufficient signal from the small region of interest defined for the voxelated TG. One way to circumvent this difficulty is to increase the size of the spectral VOI box to increase the signal. Greater signal can be obtained by temporarily removing the VSS bands and increasing the actual size of the VOI box or even by turning off the voxelated TG so that the automatic prescan may be completed to obtain an initial TG setting (Figure 6). Using this TG setting as a starting value, we can manually prescan to find a final optimal TG value (typically 190) by gradually restoring the desired scan prescriptions (e.g., the VOI size and the placement of the VSS bands).
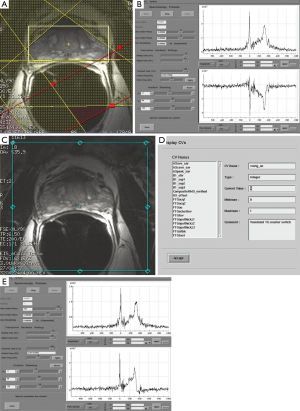
Once the prescan settings are optimized, we can acquire high-quality spectra successfully.
Discussion
In endorectal MRSI at a higher field strength, the magnetic susceptibility-related field inhomogeneity from the prostate-air/rectum interface increases proportionally. Without a good magnetic field shimming, the increased spectral separation may not directly benefit the spectral quantitation. Filling the e-coil with PFC instead of air is the first step to improve the magnetic field inhomogeneity. PFC has similar physical properties to soft tissue and has been demonstrated to improve the field inhomogeneity substantially at 1.5 T (12) as well as at 3 T.
Another complexity in 3 T MRSI is the increased RF inhomogeneity owing to the shortened RF wavelength and increased dielectric effects at the higher magnetic field strength. At 1.5 T, the RF wavelength is typically much longer than the body size. As a result, the dielectric shading is minimal and using the built-in body RF coil for the TG setting is rarely a problem. At 3 T, however, the RF wavelength becomes comparable or even shorter than a typical body size. As a result careful tuning of TG becomes critical in obtaining an acceptable spectral quality. Poorly tuned TG will result in incorrect flip angle of the RF pulses, which can lead to incomplete fat suppression. While the voxelated TG option does help reduce the sensitivity of the RF to B1-inhomogeneity, in our experience, it is not always feasible to achieve the correct TG for every patient. Therefore, careful manual tuning by observing the residual fat signal is often necessary. In the manual prescan, the tuning steps we describe above allowed us to obtain an optimal TG setting that avoids the potential pitfalls of relying on signals from the built-in body RF coil.
Our experience is based on the software of a specific vendor. However, most of the technical challenges and technical solutions we have described in optimizing 3 T prostate MRSI data acquisition should be applicable to all 3 T scanners.
In summary, the 3 T can potentially improve the spectral quality of prostate MRSI. We presented our initial experience in using 3 T MRSI prototype software and some practical steps to address several important challenges in minimizing the effect of the increased magnetic field and RF field inhomogeneity in order to obtain optimal spectral quality.
Acknowledgements
We would like to acknowledge Mr. Kelly Duggan for his editorial support of images and Ms. Jill Designe for her editorial support of the manuscript.
Funding: The work was conducted with the fund from the United States Department of Defense. (Grant Number, PC061612; Award Number, W81XWH-07-1-0110). The funding agency has no involvement in study design, in the writing of the report, and in the decision to submit the paper for publication.
Disclosure: The authors declare no conflict of interest.
References
- Thompson J, Lawrentschuk N, Frydenberg M, Thompson L, Stricker P, USANZ. The role of magnetic resonance imaging in the diagnosis and management of prostate cancer. BJU Int 2013;112 Suppl 2:6-20. [PubMed]
- Grant K, Lindenberg ML, Shebel H, Pang Y, Agarwal HK, Bernardo M, Kurdziel KA, Turkbey B, Choyke PL. Functional and molecular imaging of localized and recurrent prostate cancer. Eur J Nucl Med Mol Imaging 2013;40 Suppl 1:S48-59. [PubMed]
- Hoeks CM, Barentsz JO, Hambrock T, Yakar D, Somford DM, Heijmink SW, Scheenen TW, Vos PC, Huisman H, van Oort IM, Witjes JA, Heerschap A, Fütterer JJ. Prostate cancer: multiparametric MR imaging for detection, localization, and staging. Radiology 2011;261:46-66. [PubMed]
- Turkbey B, Pinto PA, Mani H, Bernardo M, Pang Y, McKinney YL, Khurana K, Ravizzini GC, Albert PS, Merino MJ, Choyke PL. Prostate cancer: value of multiparametric MR imaging at 3 T for detection — histopathologic correlation. Radiology 2010;255:89-99. [PubMed]
- Tanimoto A, Nakashima J, Kohno H, Shinmoto H, Kuribayashi S. Prostate cancer screening: the clinical value of diffusion-weighted imaging and dynamic MR imaging in combination with T2-weighted imaging. J Magn Reson Imaging 2007;25:146-52. [PubMed]
- Costello LC, Franklin RB. Citrate metabolism of normal and malignant prostate epithelial cells. Urology 1997;50:3-12. [PubMed]
- Romijn J. Polyamine Requirement of Prostate Cancer Cell Proliferation. In: Karr JP, Coffey DS, Smith RG, Tindall DJ. eds. Molecular and Cellular Biology of Prostate Cancer. New York: Plenum Press, 1991:103-14.
- Schipper RG, Romijn JC, Cuijpers VM, Verhofstad AA. Polyamines and prostatic cancer. Biochem Soc Trans 2003;31:375-80. [PubMed]
- Koutcher JA, Zakian K, Hricak H. Magnetic resonance spectroscopic studies of the prostate. Mol Urol 2000;4:143-52; discussion 153. [PubMed]
- Scheidler J, Hricak H, Vigneron DB, Yu KK, Sokolov DL, Huang LR, Zaloudek CJ, Nelson SJ, Carroll PR, Kurhanewicz J. Prostate cancer: localization with three-dimensional proton MR spectroscopic imaging--clinicopathologic study. Radiology 1999;213:473-80. [PubMed]
- Kobus T, Wright AJ, Scheenen TW, Heerschap A. Mapping of prostate cancer by 1H MRSI. NMR Biomed 2014;27:39-52. [PubMed]
- Choi H, Ma J. Use of perfluorocarbon compound in the endorectal coil to improve MR spectroscopy of the prostate. AJR Am J Roentgenol 2008;190:1055-9. [PubMed]
- Mattrey RF. The potential role of perfluorochemicals (PFCs) in diagnostic imaging. Artif Cells Blood Substit Immobil Biotechnol 1994;22:295-313. [PubMed]