Indocyanine green-loaded injectable alginate hydrogel as a marker for precision cancer surgery
Introduction
Laparoscopic surgery, a minimally invasive procedure, reduces the impact on the patient’s body and allows surgeons to perform operations through small holes (usually 0.5–1.5 cm long) as compared to traditional operations (usually through 24–36 cm long holes) (1). Past several decades have noticed development in laparoscopic surgery owing to advantages such as less bleeding and pain, rapid recovery rate, and shortened hospitalization period as compared with traditional open surgery (2). However, palpation of suspicious regions for tumor detection is impossible with laparoscopic operation unlike conventional surgery, and it is difficult to detect small soft lesions or polypectomy sites (3). Therefore, the incision range may vary according to the skills of the operator. To reduce this error and improve the accuracy of operation, it is imperative to preoperatively mark the surgical sites. To improve tumor detection, several marking methods have been developed, including fluoroscopy location (4), barium imaging (5), marking with metal clips (6,7), and tattooing using dyes such as methylene blue or India ink (8). However, each of these methods suffer from limitations. Intraoperative fluoroscopy is expensive and time-consuming, may need bowl distension, and increases contamination rate (9). Barium imaging demand the use of radiation (5) and may be unreliable (10). Metal clips are expensive and invisible outside, and may dislodge before or during surgical operation (5,8). Direct injection of a dye into the bowel may result in its diffusion and spilling into the peritoneal cavity and cause fat necrosis, focal perforation, or peritonitis (11,12). Therefore, development of a new surgical marker and an imaging method is desirable.
Fluorescence dyes with absorption and emission peaks in the near-infrared (NIR) wavelength range (650–900 nm) have been considered to serve as excellent agents to mark tumor sites (13). NIR fluorescence imaging allows detection of fluorescence signals in deep tissues by minimizing autofluorescence in the NIR wavelength range (14,15). Moreover, fluorescence signals may be sensitively detected using only a small amount of dye (nanomolar concentrations) in vivo (16). Indocyanine green (ICG), an FDA-approved NIR dye, is widely used as an imaging agent in medical diagnosis. However, its applications as a surgical marker are limited owing to the low fluorescence intensity resulting from the concentration-dependent coagulation, instability in aqueous solutions, and rapid diffusion at the site of injection (17).
Herein, we propose an injectable alginate hydrogel containing an ICG-human serum albumin (HSA) complex as a new surgical marker for minimally invasive surgery (Figure 1). Alginate is a polysaccharide resembling glycosaminoglycan as an extracellular matrix. It is a natural polymer extracted from brown algae; the excellent biocompatibility and hydrophilic characteristic have extended the use of alginate for biomedical applications, including soft tissue engineering and biomolecular delivery (18). As alginate is a polyanionic polymer (19), it can easily form a gel through ionic cross linking with divalent ions (e.g., Ca2+) (18,20), while it is not cleaved in mammals owing to the lack of suitable enzymes (21,22). In addition, the gelling time may be modulated by adjusting concentrations and types of ions and additives added to alginate solution (19).
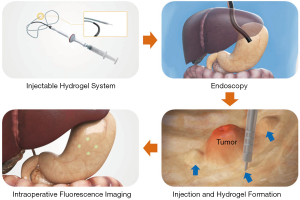
We expected the following improvements through this system. First, as ICG exhibits high affinity for proteins (23), it may easily bind to HSA and show higher fluorescence efficiency and stability than free ICG. Second, as a scaffold for the ICG-HSA complex, alginate hydrogel would prevent the rapid diffusion of the injected dye and prolong the fluorescence detection time.
Methods
Materials
Sodium alginate was obtained from KIMICA Incorporation (Tokyo, Japan, viscosity 500–600 mPa∙s). Calcium carbonate (CaCO3), D-gluconic acid (GA) solution, HSA, and ICG were purchased from Sigma-Aldrich (St. Louis, MO, USA). Dialysis membrane (MW cut-off: 50 kDa) was supplied by Spectrum Laboratories (Laguna Hills, CA, USA).
Determination of optimal conditions for ICG-HSA complex formation
The optimal concentration of ICG-HSA complex was determined. ICG (1.5 mg) was dissolved in 3 mL deionized (DI) water to obtain a concentrated ICG solution. To prepare ICG-HSA complex, 1 mL of concentrated ICG solution was added to 42.89 mg HSA at a 1:1 (ICG:HSA) molar ratio. The concentrated ICG-HSA solution was stirred at 25 °C for 1 h for stabilization. The ICG-HSA solution was then diluted with DI water to 2–100 µM range. Fluorescence spectra of ICG-HSA solutions (λex. =720 nm, λem. =740–850) were measured with a multifunctional microplate reader (Safire 2, Tecan, Männedorf, Switzerland).
The optimal ratio of ICG versus HSA to obtain maximum fluorescence intensity was evaluated. ICG concentration in DI water was fixed to 30 µM, and ICG was mixed with various molar ratios of HSA. The fluorescence spectra of the solution (λex. =720 nm, λem. =740–850) were obtained and compared.
Analysis of gelation time for alginate hydrogels containing CaCO3-GA
Sodium alginate was dissolved in DI water, dialyzed for 2 days (MWCO =50 kDa), and then freeze dried.
Sodium alginate in DI was mixed with different amounts of CaCO3 to obtain solution A (see Table 1 for the concentration of sodium alginate and CaCO3). Solution B was prepared by mixing sodium alginate solution with different concentrations of GA solution. The molar ratio of GA to CaCO3 was adjusted to 2 for the neutralization of the pH of the final solution (22). Solutions A (0.35 mL) and B (0.35 mL) were separately charged into a dual syringe and simultaneously injected into a 5 mL glass vial to prepare an in situ gel. Sol-gel transition time of alginate/Ca2+-GA composite solutions was determined by the test-tube inverting method (24) by inverting the tube every 1 min after injection. The solution state was determined by flow (sol) and non-flow state (gel).
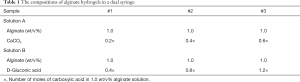
Full table
In vivo evaluation of alginate hydrogels in a mouse model
The potential utility of ICG-HSA-loaded alginate hydrogel as a surgical marker was evaluated in a mouse model. ICG, ICG-HSA solution, and ICG-HSA-loaded alginate/Ca2+-GA composite system (alginate hydrogel) were subcutaneously injected into male nude mice (6 weeks, n=3 per group). The final concentrations of sodium alginate, ICG-HSA, CaCO3, and GA in the injected gel were 1 wt/v %, 30 µM, 0.3×, and 0.6×, respectively. The injection volume of the sample solution was 100 µL. NIR fluorescence images (λex. =780 nm, λem. =845 nm) of mice were obtained using the IVIS Lumina XR system (Xenogen Corp., Alameda, CA, USA) at different time points for 8 days. The animal experiment protocol was approved by the Institutional Animal Care and Use Committee (IACUC) of the National Cancer Center (approval No. NCC-18-451) of Korea.
For quantitative analysis of fluorescence intensities in the region of interest (ROI), target-to-background ratio (TBR) and area of ICG fluorescence were determined using an imaging software. TBR was determined as follows: TBR = ROI (target)/ROI (background) (25).
In vivo evaluation of alginate hydrogels in a porcine model
A healthy male landrace pig (45 kg) was obtained from Cronex Co., Ltd. (Hwaseong, Korea). All experiments were conducted in KNOTUS Co., Ltd (Incheon, Korea) and approved by the IACUC of KNOTUS (approval No. 19-KE-358).
The pig was fed with a semifluid diet for 48 h prior to experiment inception and fed with a polyethylene glycol solution on the day prior to endoscopy. General anesthesia was administered with an injection of Zoletil 50 (VIRBAC, France, 5 mg/kg) and xylazine (Rompun®, Bayer AG, Germany, 2.5 mg/kg). The pig was intubated and anesthesia was maintained with 1–2% isoflurane added to the oxygen gas by a ventilator. The pig was administered a 50-cc saline enema through the anus until all residual fecal material was evacuated. An endoscope with an attached cap was advanced into the stomach of the pig.
ICG-HSA-loaded alginate solution was then injected into three sites (1 mL solution per site) of the submucosal space in the porcine stomach via catheter (23G needle Clear-Jet injector, Finemedix, Daegu, Korea). To mark injection sites, hemostatic clips were clipped right beside the injection sites. After 3 days of hydrogel injection, a fluorescent laparoscopic system was installed on the abdomen of the pig, and the fluorescence signal generated from the fluorescence surgical marker located inside the stomach was evaluated in real time using a fluorescence laparoscope system. The pig was euthanized, and the stomach was dissected to verify that the position identified as the gel injection site by the fluorescence image was accurate (26).
NIR fluorescence laparoscopic system
Fluorescence light source is composed of white and infrared light (Figure 2). White light has a color temperature of 5,500 K at a maximum output of 2,600 lm and an infrared wavelength of 785 nm at a maximum output of 3.5 W. Two light sources are mixed with a 5 mm diameter fiber bundle and irradiated onto the image target using a laparoscope (λex. =785 nm, λem. =805 nm). Laparoscopes are designed to target images 50 mm apart with a 10 mm diameter and 70° field of view.
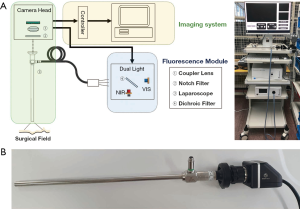
The eyepiece of the laparoscope was attached to a camera head to simultaneously acquire color and fluorescence images. The camera head comprised a coupler lens and an image-accepting sensor. The coupler lens clarifies the focus of the image targeted by the laparoscope and delivers the image to the sensor. The image-accepting sensor passes the data to an imaging system for image construction.
Results
Optimal condition for ICG-HSA complex formation
To determine the optimal concentration of ICG-HSA, fluorescence spectra were obtained after mixing ICG and HSA at different concentrations and mole ratios. The ICG-HSA complex (1:1 mole ratio) showed a continuous increase in the fluorescence intensity in the concentration range of 2–30 µM (Figure 3A,B). The maximum fluorescence intensity remained unchanged above 30 µM. Further, the change in the fluorescence intensity of ICG-HSA complex was analyzed by varying the mole ratio of HSA to ICG (from 0 to 2; Figure 3C,D). As a result, the fluorescence intensity of the complex solution increased as the amount of HSA in the ICG-HSA complex increased. In particular, the fluorescence signal increased until the two substances were combined at a one-to-one ratio, while only a little change was observed with any further increase in HSA. Therefore, we selected the one-to-one combination of ICG-HSA in subsequent experiments.
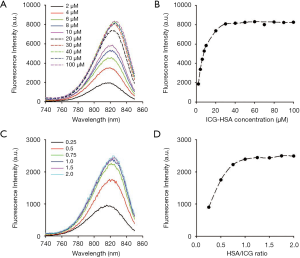
Gelation time of alginate hydrogels containing CaCO3-GA
To prepare alginate hydrogel with an adequate gelation time as a surgical marker, different concentrations of calcium ions were mixed with the alginate solution using a dual syringe (Table 1). Gelation time of the alginate solution was evaluated.
As shown in Figure 4A, the alginate solution containing 0.1× calcium ion remained in its liquid state (sol) for about 46 min after injection before forming a solid gel. Under 0.2× calcium concentration, the solution was in a slightly liquid state (sol) immediately after injection and changed to the solid gel state after about 2 min. The solution exposed to 0.3× calcium concentration formed a weak gel immediately after injection and changed to a solid gel state after about 1 min. The gelation time is shown in Figure 4B according to the final concentration of calcium ions in the injected gel. The gelation time of alginate was shortened as the concentration of calcium ion increased from 0.1× to 0.3×. In particular, the alginate solution treated with 0.3× calcium existed in a solid state (gel) immediately after injection. Therefore, 0.3× calcium concentration was selected for further experiments.
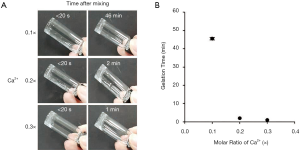
In vivo evaluation of alginate hydrogels in a mouse model
We next evaluated the ICG-HSA-loaded alginate hydrogel as a surgical marker in vivo. The aqueous solutions of ICG, ICG-HSA, ICG-HSA-loaded alginate were subcutaneously injected into nude mice using a dual syringe (Figure 5A). Then, NIR fluorescence images and photographs of the mice were periodically obtained (Figure 5B,C). As a result, the fluorescence intensities at ICG and ICG-HSA injection sites rapidly decreased with time, and most of the fluorescence signals disappeared after 24 h from injection. Quantitative analyses of the fluorescence intensity and area of fluorescence emission with time (Figure 6) showed that most of the injected ICG and ICG-HSA complex rapidly diffused into the surrounding tissues and disappeared from the injection sites within 24 h. Only 16% and 12% fluorescence signals were retained at ICG and ICG-HSA injection sites, respectively, relative to the signal detected at the start. In contrast, the mice receiving alginate hydrogel retained 61% fluorescence signal 48 h post-injection, while significant NIR fluorescence persisted at the injection sites for about 4 days. The area of fluorescence emission was maintained at low values for several days, indicating that the alginate hydrogel effectively prevented the diffusion of the ICG-HSA complex from the injection site.
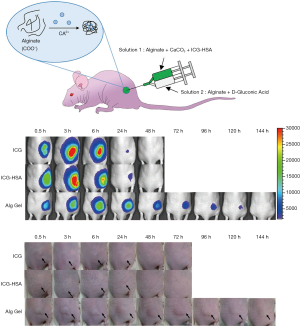
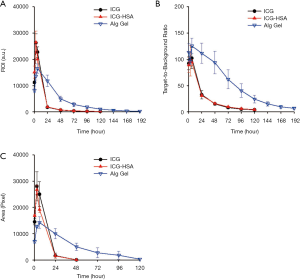
Figure 5C shows the images of the injection site of ICG, ICG-HSA solution, and alginate hydrogel in nude mice. In particular, the injection sites for ICG and ICG-HSA solutions appeared slightly swollen immediately after injection, and the solutions spread and became invisible after about 24 h. On the other hand, protrusions formed immediately after injection in the alginate hydrogel-treated mice, and were retained for 5 days.
In vivo evaluation of alginate hydrogels in a porcine model
The potential application of ICG-HSA-loaded alginate hydrogel as a surgical marker was evaluated in a porcine model. The solution was injected into the submucosal space of the porcine stomach using an injector. Hemostatic clips were clipped right beside the injection sites. After 3 days from injection, NIR fluorescence imaging was performed for the injected sites using a fluorescence laparoscopic system (Figure 7A). As a result, the location and margins of injection sites could be clearly distinguished in real time using NIR fluorescence images (Figure 7B).
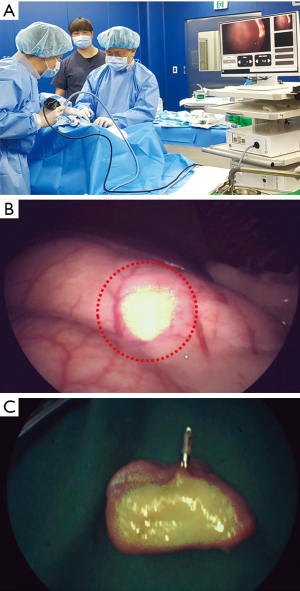
To ensure that the fluorescence signal was emitted from the injected hydrogel, porcine stomach was opened and the fluorescence was evaluated ex vivo (Figure 7C). Strong fluorescence signal was detected right beside the hemostatic clip, confirming that the NIR fluorescence signal detected using fluorescence laparoscopic system was derived from the injection sites of ICG-HSA-loaded alginate hydrogels.
Discussion
The incidence of early gastrointestinal cancers has been increasing, resulting in the increase in popularity of the use of the laparoscopic system for related surgeries. However, real-time detection of small lesions is difficult due to the lack of tactile sensation using the laparoscopic system. Therefore, ICG-HSA-loaded alginate hydrogels were developed as a novel surgical marker for the non-invasive and real-time detection of cancer sites, enabling precision cancer surgery.
As depicted in Figure 5B, the ICG-HSA complex initially emits strong fluorescence signals at the injection site; however, the signals rapidly decrease within 24 h due to dye diffusion from the injection site to the surrounding tissues. Alginate was mixed with both ICG-HSA and an ion crosslinker (e.g., Ca2+) to form a hydrogel, successfully inhibiting the diffusion of the ICG dyes from the injection site. Because the solution is injected through a catheter, it is essential to form a gel after injection to prevent jamming. Calcium chloride (CaCl2) has been widely used for the preparation of hydrogels; however, gel formation after CaCl2 addition is excessively rapid. It is also difficult to form a uniform gel and the injector line gets easily clogged. Therefore, calcium carbonate (CaCO3) was used in this study as a source of calcium ions to delay the gelation time (21). GA in solution B was added to facilitate CaCO3 dissolution at the injection site.
In vivo animal studies in mouse and porcine models confirmed that dye diffusion from the injection sites could be effectively prevented using the ICG-HSA-loaded alginate hydrogel. Thus, strong fluorescence at the injection sites was maintained for more than 3 days, while the fluorescence emission area remained narrow.
In conclusion, here we demonstrate the application of ICG-HSA-loaded alginate hydrogel as a new surgical marker for noninvasive and real-time identification of tumor sites. In vivo animal studies in mouse and porcine models revealed promising results of this hydrogel system for prevision surgery of gastrointestinal tumors. This hydrogel system is expected to mark lesions using various contrast agents and serve as a drug delivery system following incorporation of suitable therapeutics.
Acknowledgments
Funding: This work was supported by the Ministry of Oceans and Fisheries (the project title: Development of marine material based near infrared fluorophore complex and diagnostic imaging instruments) and also by the National Cancer Center (1910070), Republic of Korea.
Footnote
Conflicts of Interest: The authors have no conflicts of interest to declare.
Open Access Statement: This is an Open Access article distributed in accordance with the Creative Commons Attribution-NonCommercial-NoDerivs 4.0 International License (CC BY-NC-ND 4.0), which permits the non-commercial replication and distribution of the article with the strict proviso that no changes or edits are made and the original work is properly cited (including links to both the formal publication through the relevant DOI and the license). See: https://creativecommons.org/licenses/by-nc-nd/4.0/.
References
- Lu L, Zhou D, Jian X, Deng J, Yang P, Ding W. Laparoscopic colorectomy for colorectal cancer: retrospective analysis of 889 patients in a single center. Tohoku J Exp Med 2012;227:171-7. [Crossref] [PubMed]
- Koh YX, Goh BKP. Minimally invasive surgery for gastric gastrointestinal stromal tumors. Transl Gastroenterol Hepatol 2017;2:108. [Crossref] [PubMed]
- Aboosy N, Mulder CJ, Berends FJ, Meijer JW, Sorge AA. Endoscopic tattoo of the colon might be standardized to locate tumors intraoperatively. Rom J Gastroenterol 2005;14:245-8. [PubMed]
- Rogers BH. Colonoscopy with fluoroscopy. Gastrointest Endosc 1990;36:71-2. [Crossref] [PubMed]
- Choi Y, Kim KG, Kim JK, Nam KW, Kim HH, Sohn DK. A novel endoscopic fluorescent clip for the localization of gastrointestinal tumors. Surg Endosc 2011;25:2372-7. [Crossref] [PubMed]
- Tabibian N, Michaletz PA, Schwartz JT, Heiser MC, Dixon WB, Smith JL, Graham DY. Use of an endoscopically placed clip can avoid diagnostic errors in colonoscopy. Gastrointest Endosc 1988;34:262-4. [Crossref] [PubMed]
- Ohdaira T, Konishi F, Nagai H, Kashiwagi H, Shito K, Togashi K, Kanazawa K. Intraoperative localization of colorectal tumors in the early stages using a marking clip detector system. Dis Colon Rectum 1999;42:1353-5. [Crossref] [PubMed]
- Fu KI, Fujii T, Kato S, Sano Y, Koba I, Mera K, Saito H, Yoshino T, Sugito M, Yoshida S. A new endoscopic tattooing technique for identifying the location of colonic lesions during laparoscopic surgery: a comparison with the conventional technique. Endoscopy 2001;33:687-91. [Crossref] [PubMed]
- Cohen JL, Forde KA. Intraoperative colonoscopy. Ann Surg 1988;207:231-3. [Crossref] [PubMed]
- Frager DH, Frager JD, Wolf EL, Beneventano TC. Problems in the colonoscopic localization of tumors: continued value of the barium enema. Gastrointest Radiol 1987;12:343-6. [Crossref] [PubMed]
- Coman E, Brandt LJ, Brenner S, Frank M, Sablay B, Bennett B. Fat necrosis and inflammatory pseudotumor due to endoscopic tattooing of the colon with india ink. Gastrointest Endosc 1991;37:65-8. [Crossref] [PubMed]
- Park SI, Genta RS, Romeo DP, Weesner RE. Colonic abscess and focal peritonitis secondary to india ink tattooing of the colon. Gastrointest Endosc 1991;37:68-71. [Crossref] [PubMed]
- Weissleder R, Ntziachristos V. Shedding light onto live molecular targets. Nat Med 2003;9:123-8. [Crossref] [PubMed]
- Müller MG, Georgakoudi I, Zhang Q, Wu J, Feld MS. Intrinsic fluorescence spectroscopy in turbid media: disentangling effects of scattering and absorption. Appl Opt 2001;40:4633-46. [Crossref] [PubMed]
- Sheng Z, Hu D, Zheng M, Zhao P, Liu H, Gao D, Gong P, Gao G, Zhang P, Ma Y, Cai L. Smart human serum albumin-indocyanine green nanoparticles generated by programmed assembly for dual-modal imaging-guided cancer synergistic phototherapy. ACS Nano 2014;8:12310-22. [Crossref] [PubMed]
- Ntziachristos V, Ripoll J, Wang LV, Weissleder R. Looking and listening to light: the evolution of whole-body photonic imaging. Nat Biotechnol 2005;23:313-20. [Crossref] [PubMed]
- Saxena V, Sadoqi M, Shao J. Degradation kinetics of indocyanine green in aqueous solution. J Pharm Sci 2003;92:2090-7. [Crossref] [PubMed]
- Deepthi S, Jayakumar R. Alginate nanobeads interspersed fibrin network as in situ forming hydrogel for soft tissue engineering. Bioact Mater 2017;3:194-200. [Crossref] [PubMed]
- Andersen T, Auk-Emblem P, Dornish M. 3D Cell Culture in Alginate Hydrogels. Microarrays (Basel) 2015;4:133-61. [Crossref] [PubMed]
- Somo SI, Langert K, Yang CY, Vaicik MK, Ibarra V, Appel AA, Akar B, Cheng MH, Brey EM. Synthesis and evaluation of dual crosslinked alginate microbeads. Acta Biomater 2018;65:53-65. [Crossref] [PubMed]
- Lee KY, Mooney DJ. Alginate: properties and biomedical applications. Prog Polym Sci 2012;37:106-126. [Crossref] [PubMed]
- Bidarra SJ, Barrias CC, Granja PL. Injectable alginate hydrogels for cell delivery in tissue engineering. Acta Biomater 2014;10:1646-62. [Crossref] [PubMed]
- Liberale G, Vankerckhove S, Caldon MG, Ahmed B, Moreau M, Nakadi IE, Larsimont D, Donckier V, Bourgeois P, Group R. D for the Clinical Application of Fluorescence Imaging of the Jules Bordetʼs Institute. Fluorescence Imaging After Indocyanine Green Injection for Detection of Peritoneal Metastases in Patients Undergoing Cytoreductive Surgery for Peritoneal Carcinomatosis From Colorectal Cancer: A Pilot Study. Ann Surg 2016;264:1110-1115. [Crossref] [PubMed]
- Shinde UP, Moon HJ. Ko du Y, Jung BK, Jeong B. Control of rhGH Release Profile from PEG-PAF Thermogel. Biomacromolecules 2015;16:1461-9. [Crossref] [PubMed]
- Klohs J, Steinbrink J, Nierhaus T, Bourayou R, Lindauer U, Bahmani P, Dirnagl U, Wunder A. Noninvasive near-infrared imaging of fluorochromes within the brain of live mice: an in vivo phantom study. Mol Imaging 2006;5:180-7. [Crossref] [PubMed]
- Hyun JH, Kim SK, Kim KG, Kim HR, Lee HM, Park S, Kim SC, Choi Y, Sohn DK. A novel endoscopic fluorescent band ligation method for tumor localization. Surg Endosc 2016;30:4659-63. [Crossref] [PubMed]