Differences in functional brain alterations driven by right or left facial nerve efferent dysfunction: Evidence from early Bell’s palsy
Introduction
Peripheral facial palsy is a condition in which facial nerve efferent dysfunction results in an inability to control the facial muscles on the affected side. Approximately 80% of the cases of facial nerve paralysis is idiopathic and unilateral and thus define as Bell’s palsy (1,2). The facial nerve is the main motor pathway to the facial muscles and is involved in regulating facial expression, movement, and other functions (3,4). Bell’s palsy is characterized by asymmetric motor conduction of facial nerve branches and imbalance of facial muscles movement, which may result in a substantial psychological impact on the patient and trigger brain cortical reorganization (5). Thus, research on brain function alterations related to this condition will facilitate the understanding of the mechanisms of functional integration within the cerebral cortex (6).
Functional magnetic resonance imaging (fMRI) is an advanced technique, widely applied in the evaluation of numerous diseases, including peripheral facial palsy (7,8). An interesting fMRI approach involves imaging the brain neural network by examining functional connectivity (FC) when the individual does not focus on external stimuli, and the brain is at wakeful rest (9-11). Conducting fMRI of brain activity in the resting state requires less patient cooperation compared with the same analysis during complex activation tasks. Previous studies on Bell’s palsy have often focused on task-fMRI, which indicated that some cortical functional connectivities are interrupted in the early phase, with an increase in motor integration. Functional integration mainly occurs in the hemisphere contralateral to the paralyzed side (12-14). The FC between the significant motor cortex and other related brain regions gradually recovered to the average level when facial nerve function was gradually recovered (15-17).
The resting-state fMRI (R-fMRI) offers a wealth amount of helpful information for detecting functional brain changes and interpret the results of task-fMRI, as the brain is somewhat active even when no task is being performed (18,19). Both the amplitude of low-frequency fluctuation (ALFF) and the fractional amplitude of low-frequency fluctuation (fALFF) can reflect the spontaneous activity of the brain from the perspective of brain energy metabolism in R-fMRI (20). fALFF is more sensitive and specific than ALFF at low frequencies and can more accurately reflect the strength of spontaneous activity in the brain (21). FC is another commonly used quantification method, defined as the temporal series correlation among corresponding functionally activated events (22). However, research using R-fMRI in patients with peripheral facial palsy is still in its initial stages (23). Recent studies have revealed that in patients with facial paralysis, the FC between the ipsilateral anterior cingulate gyrus and other related brain areas is enhanced, and such enhancement is related to cortical reconstruction during rehabilitation (24). Changes in the default mode network (DMN) have been linked to the altered brain state in the patients with Bell’s palsy. The DMN is the distinct brain network connecting the regions that are most active when the brain is at rest, such as the medial temporal lobe, medial prefrontal cortex, and posterior cingulate cortex, known to be highly correlated with each other (25). In patients with facial nerve paralysis, the functional connectivities of the regions in the DMN show changes depending on the pathological states, which may involve various interacting brain regions related to sensory, motor, emotion, and cognitive functions (25). However, for patients with right or left early stage Bell’s palsy, studies on the bilateral differences in abnormal functional activities using fALFF and FC are rare. The association of altered FC with clinical presentations is not yet clear. Investigation of patients with different-sided Bell’s palsy in the early stage would be helpful to gain insight into the different mechanisms involved in functional integration driven by unilateral facial efferent nerve dysfunction and to provide a neuroimage foundation for a suitable treatment strategy to be implemented as early as possible in the course of the disease.
In the current study, we hypothesized that several abnormal brain intrinsic functional activities in various brain regions would be found in patients with early-stage right or left Bell’s palsy. Furthermore, since score criteria regarding the clinical presentation were shown to be important in rating facial nerve injury and disability, we also hypothesized that they would partially reveal the differences between patients and healthy controls. We tested this hypothesis using R-fMRI to examine the fALFF, and the brain areas with abnormal fALFF were selected as seeds in the following FC analysis. Besides, correlations between altered FC and clinical presentation scores were analyzed in the left and right palsy groups.
Methods
Subjects
The Ethics Committee of our hospital approved this study and written informed consent was obtained from all patients before the study began. Sixty-seven patients were enrolled. The inclusion criteria were: (I) first-ever idiopathic unilateral facial nerve palsy (two physicians confirmed the diagnosis); (II) right-handed; and (III) onset time of 2–7 days. Patients with a history of neurologic, metabolic, or psychiatric disorders, or who were using psychotropic drugs, were excluded. The patients were divided into a right facial paralysis group (34 cases, 13 men and 21 women, mean age 47.27±12.96 years) and left facial paralysis group (33 cases, 12 men and 21 women, mean age 48.11±13.27 years). Thirty-seven healthy controls (14 men and 23 women, mean age 46.05±13.65 years) were recruited from graduate students, the local community, and the hospital staff. The inclusion criteria were: (I) no history of neurologic, metabolic, or psychiatric diseases, and not currently using psychotropic drugs; (II) no family history of neurological diseases; (III) normal conventional head MRI examination results; (IV) Toronto Facial Grading System (TFGS) (26) scores of 100 points; and (V) being right-handed. The TFGS score was evaluated for each participant.
MRI data acquisition
Participants were required to rest in a supine position with their eyes closed, breath regularly, and to minimize head movements. Also, they were asked to remain awake and not to think, and to wear rubber earplugs to reduce noise. We used a 3.0 T MR imaging system (GE healthcare, Discovery MR750, Milwaukee, WI, USA) with a supporting head quadrature coil. The parameters of axial resting state blood oxygen-level dependent-fMRI were: repetition time (TR) =2,000 ms, echo time (TE) =30 ms, slice thickness =3.5 mm, slice spacing =0.7 mm, field of view (FOV) =240 mm × 240 mm, matrix =64×64, flip angle =90°, and number of excitations (NEX) =1, 34 slices and 240 phases; The parameters of sagittal 3D T1 weighted image with three-dimensional fast spoiled gradient-echo sequences (3D FSPGR) were: TR =6.7 ms, TE = min full, slice thickness =1.0 mm, FOV =256 mm × 256 mm, acquisition matrix =256×256, and NEX =1.
Data processing and analysis
Data pre-processing
The Data Processing Assistant for Resting-State fMRI (DPARSF, Advanced Edition) software was used (27). The preprocessing procedure was as follows: First, the data format was converted from DICOM to NIFTI. Next, the first ten time points were removed, slice-timing correction and head motion correction (standard 2.0 mm or 2°) were performed, and the head movement parameters were extracted from all participant data. Spatial normalization was then achieved through T1 anatomical images with unified segmentation. Spatial smoothing was conducted with the Full Width at Half Maximum set at 4, and linear drift was removed. Covariate regression was then carried out [the white matter and cerebrospinal fluid (CSF) region signals were extracted, linear fitting was conducted on the time series signals in the white matter and CSF regions, and the signals of the white matter and CSF were removed from the overall signal]. Finally, fALFF calculation and band-pass filtering (frequencies set as 0.01–0.08 Hz), to remove the effects of high-frequency noise and low-frequency drift, were performed.
fALFF and FC analysis
We employed the fALFF value as an index to analyze the R-fMRI data. The time series of each voxel obtained from preprocessed data was converted to the frequency domain using a fast Fourier transform, and the square root of the power spectrum was computed as the ALFF. The ALFF value was further divided by the global mean ALFF value to standardize the data within each group. Then, the normalized ALFF value was divided by the ALFF value of the entire band to obtain the normalized fALFF value of each voxel. The fALFF values were compared between each pair of groups to select the region of interest (ROI) to be used in the following FC computation.
The peak coordinates of the regions with altered fALFF values were used as the centers of 6-mm spherical seed brain ROIs, created with the wfu_pickatlas tool of the SPM12 software (28). We computed the time serial correlation coefficient between every pair of ROIs. Then, we computed the mean overall FC of each brain region with the other brain regions. The areas of the bilateral middle cingulate cortex (MCC), altered in the comparison between right and left palsy groups, were in close contact and were considered a unified larger region in the FC analysis because of their unclear boundaries.
Statistical analyses
The demographic and clinical data of the study population were analyzed with SPSS (version 23.0, IBM Corp., Armonk, NY, USA). The age and education level among the three groups were compared by one-way analysis of variance (ANOVA). The palsy duration and TFGS scores were compared, by two-sample t-tests, only between the right and left Bell’s palsy groups. The chi-squared test was used to compare the sex ratio among the three groups. A one-way analysis of covariance (ANCOVA) was applied to compare the differences in fALFF among the three groups with age, sex, education level, and head movement parameters as covariates. Post hoc t-tests were performed to identify the inter-group differences between right palsy and healthy control groups, left palsy and healthy control groups, and right and left palsy groups. The false discovery rate (FDR) correction was used for multiple comparisons in the fMRI data analysis with a threshold of 0.05 (29).
Fisher z-values were extracted from the correlation coefficients by Fisher’s transformation, and used in multiple regression across all patients, with age, sex, and education level set as confounding covariates. A two-sample t-test was used to compare the means of the overall FC of each ROI with other regions between the right palsy and healthy control groups, left palsy and healthy control groups, and right and left palsy groups. Also, the difference in the FC of every pair of ROIs was analyzed between each pair of groups. FDR correction was performed with a threshold of 0.05. A two-tailed Pearson correlation analysis was performed between the TFGS scores of the patients in each palsy group and the corresponding functional connectivity between paired ROIs, and P values <0.05 were considered statistically significant.
Results
Clinical data
The results indicated no significant differences in sex, age, and education level among the two patient groups and the control group (P>0.05). The time from onset to examination was set to 0, and the TFGS score was equal to 100 for the healthy controls. The palsy duration (mean ± standard deviation: right palsy, 4.52±2.46 days; left palsy, 4.83±2.14 days) and TFGS scores (mean ± standard deviation: right palsy, 17.23±16.22; left palsy, 18.71±16.09) were compared between the right and left patient groups, and no significant differences were found (P>0.05) (Table 1).
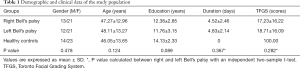
Full table
Differences among the three groups
A significant statistical difference in fALFF was found among the right palsy, left palsy, and healthy control groups, and the post hoc t-tests revealed significant differences between all three group pairs (Table 2).
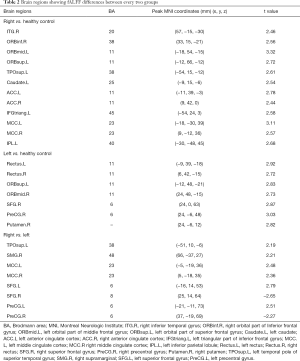
Full table
Differences between the right palsy and healthy control groups
Compared with healthy controls, the right Bell’s palsy group showed significantly increased fALFF values in the right inferior temporal gyrus (ITG), right orbital part of inferior frontal gyrus (ORBinf), left orbital part of the middle frontal gyrus (ORBmid), left orbital part of the superior frontal gyrus (SFG) (ORBsup), left temporal pole of the superior temporal gyrus (TPOsup), left caudate, bilateral anterior cingulate cortex (ACC), left triangular part of the inferior frontal gyrus (IFGtriang), bilateral MCC, and left inferior parietal gyrus (IPL) (Table 2).
Comparing the right palsy group with the healthy controls group, the means of the overall inter-regional FC of each brain region with other regions showed no significant differences (Table 3). In the right palsy group, the brain regions pairs with enhanced functional connectivities were the right ITG and orbital part of inferior frontal gyrus (P<0.05); right ITG and left MCC (P<0.05); left ORBmid and bilateral anterior cingulate cortex (P<0.05 and P<0.01); left caudate and bilateral anterior cingulate cortex (P<0.05); left and right MCC (P<0.01), and left MCC and inferior parietal gyrus (P<0.01). Also, the only region pair with decreased FC was the right ITG and left orbital part of middle frontal gyrus (P<0.01) (Figure 1, Table S1).
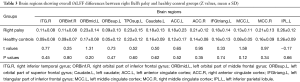
Full table
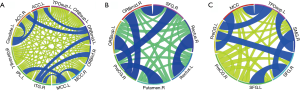
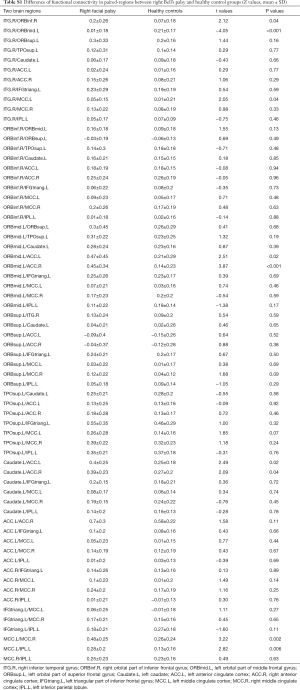
Full table
Differences between the left palsy and healthy control groups
The left palsy group showed significantly increased fALFF, compared with the healthy controls, in the left and right rectus, left orbital part of the SFG, right orbital part of middle frontal gyrus, right SFG, right precentral gyrus (PreCG), and right putamen (Table 2).
Comparing the left palsy group with the healthy controls, the means of the overall inter-regional functional connectivities of each brain region with other regions showed no significant differences (Table 4). In the left facial palsy group, the brain regions pairs with enhanced FC were the left and right rectus (P<0.05); left orbital part of SFG and right PreCG (P<0.01); right orbital part of middle frontal gyrus and SFG (P<0.05); right orbital part of middle frontal gyrus and PreCG (P<0.01); right SFG and PreCG (P<0.01), and right PreCG and putamen (P<0.05) (Figure 1B, Table S2).
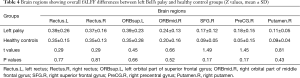
Full table
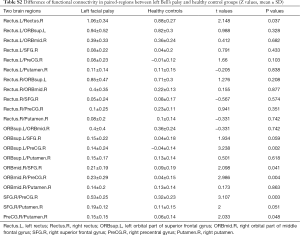
Full table
Differences between the right and left palsy groups
The right Bell’s palsy group showed significantly increased fALFF values, compared with the left palsy group, in the left temporal pole of the TPOsup, right supramarginal gyrus (SMG), bilateral MCC, left SFG, and left PreCG, and significantly decreased fALFF values in the right SFG and PreCG (Table 2, Figure 2).
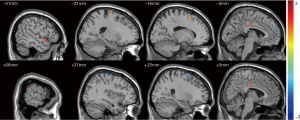
The mean overall inter-regional functional connectivities of each brain region showed significant differences between the right and left palsy groups. Increased functional connectivities in the right palsy group were observed in the right SMG (P<0.01), bilateral SFG (P<0.05), and left PreCG (P<0.01) (Table 5, Figure 3).
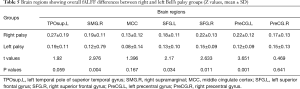
Full table
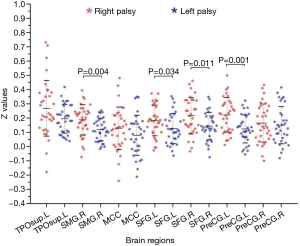
Compared with that of the left palsy group, the region pairs in the right palsy group showing increased functional connectivities were the left temporal pole of the TPOsup and right SMG (P<0.05), left temporal pole of the TPOsup and MCC (P<0.05), left temporal pole of the TPOsup and PreCG (P<0.05), right SMG and SFG (P<0.05), MCC and left PreCG (P<0.05), left and right SFG (P<0.05), and right SFG and left PreCG (P<0.05) (Figure 1C, Table S3).
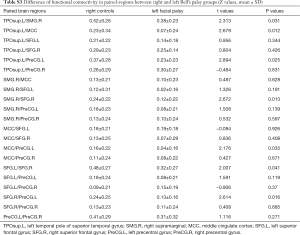
Full table
Correlation analysis
In the right palsy group, the FC of brain region pairs that showed a negative correlation with the TFGS score was the left temporal pole of the TPOsup and PreCG (P<0.05), while the right SFG and left PreCG showed a positive correlation (P<0.01).
In the left palsy group, the functional connectivities of the brain region pairs showing a negative correlation with the TFGS were the left temporal pole of the TPOsup and right SMG (P<0.01), right SMG and SFG (P<0.01) (Table S4, Figure 4).
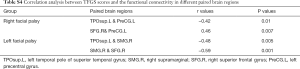
Full table
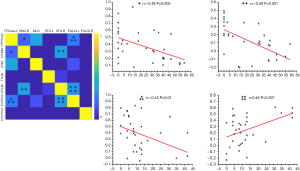
Discussion
We identified different brain functional remodeling mechanisms driven by right or left facial nerve efferent dysfunction in unilateral Bell’s palsy. Compared with the healthy controls, the right palsy group showed significantly increased fALFF values in the right ITG, right ORBinf, left orbital part of middle frontal gyrus, and orbital part of SFG, bilateral cingulate cortex, and left triangular part of inferior frontal gyrus. These are all related to emotion control, including depression, anxiety, and other negative states (30-32). Some of these regions were also identified in the left palsy group. Furthermore, compared with the healthy controls, only the right palsy group showed increased fALFF values in the left TPOsup and IPL, involved in emotion perception and sensory (neural?) transmission (33,34). Also, the left caudate nucleus, identified in the right palsy group, is associated to motor function (35), while the left palsy group showed increased fALFF values in the right SFG, PreCG, and putamen, involved in the remodeling of the motor network (36). These differences suggest that the bilaterally different brain functional alterations derive from asymmetrical compensation in the left and right hemispheres (37). However, the mean overall inter-regional FC of each region showed no significant differences in either the right or left palsy groups compared with the healthy controls. This indicates that the brain functional activities in patients with facial palsy serve mainly to compensate for the damaged function, thereby maintaining the balance of the overall connectivity strength among these related functional regions. The brain region pairs with enhanced functional connectivities in the right palsy group compared with healthy controls were mainly related to emotion processing and perception functions; on the other hand, the left palsy group showed brain region pairs with enhanced FC mainly involved in motor function remodeling. These regions with altered FC further highlight the differences in functional reorganization activities, mainly involving the emotional processes network in the right palsy group, and motor-related functional integration in the left palsy group, compared to the healthy controls. We speculate that psychological stress and negative emotions are heightened to different degrees in patients with bilateral Bell’s palsy, because of their involuntary abnormal facial expressions and movements (30).
Compared with the results of the left palsy group, the right palsy group showed significantly increased fALFF values in the left temporal pole of the TPOsup, right SMG, bilateral MCC, left SFG, and left PreCG, and decreased fALFF in the right SFG and PreCG. Among these regions, the cingulate cortex is associated with emotional processing and involved in depression and anxiety (25,31,32,38); abnormal function in patients with Bell’s palsy in this region has also been found by previous studies (37). This bilateral difference probably reflects different mechanisms of functional integration of emotions in patients with different side palsy (31). Compared with the results of the left Bell’s palsy group, the right Bell’s palsy group showed decreased fALFF values in the right SFG and PreCG, involved in the remodeling of the motor network (39). This indicates that motor function reintegration in patients with left and right facial palsy is different.
Interestingly, the right palsy group showed increased overall FC in the right SMG, bilateral SFG, and left PreCG compared with that of the left palsy group. Most of these regions are involved in the functional integration of motor and sensory transmission. Also, the SFG is related to self-awareness and laughter and is known as an essential gray matter nucleus with reported abnormalities in patients with Bell’s palsy (22,23,36). Compared with the results of left Bell’s palsy group, the right Bell’s palsy groups showed increased fALFF values in the left temporal pole of the TPOsup and right SMG, which play vital roles in sensory transmission (33,34,40). This finding suggests that afferent nerve function is enhanced in the brains of patients with right early facial palsy, in agreement with earlier findings (28,41). This bilaterally different sensory reintegration mechanism probably derives from asymmetrical compensation in the bilateral hemispheres (37).
Furthermore, the region pairs with lower FC in the left palsy group highlighted the differences in the functional reorganization activities involved in emotional processes, motor networks and sensory transmission functions (24). We also found that all the altered functional connectivities of region pairs in the right palsy group were significantly stronger than those in the left palsy group, suggesting a higher-level regulation of the dysfunction or damage in the early palsy stage (37). The exact remodeling mechanism remains unclear in the recovery stage, and further investigation is warranted.
The brain region pairs showing a negative correlation with the TFGS scores in patients with right facial palsy were the left temporal pole of TPOsup and left PreCG, and right SFG and left PreCG. On the other hand, the brain region pairs showing negative correlations with the TFGS scores in patients with left facial palsy were the left temporal pole of the TPOsup and right SMG, and right SMG and right SFG. Since the functional integration of most of these regions is related to sensory transmission and, to a lesser extent, to motor regulation (38), we speculate that the increase of motor regulation accompanies the increase of disease severity and that sensory transmission changes in patients with early facial palsy are related to disease severity. Thus, exercise rehabilitation therapy, which could increase facial muscles stimulation and nerve transmission actions, should be given more prominence in the treatment of patients with facial palsy, as was also suggested in previous studies (42,43).
This study has some limitations. Because of the difficulty in performing imaging examinations in patients with facial paralysis in the early stage, the sample size was relatively small, and we did not conduct follow-up or dynamic observations. Besides, since we only focused on local FC changes of selected regions, our study could not show inter-cortical connectivity changes in the whole brain. In a future study, the number of cases will be increased so that patients can be stratified by disease stage, and more comprehensive network analysis will be performed.
Conclusions
Brain reintegration mechanisms appear to be differently driven by right or left facial nerve efferent dysfunction in patients with Bell’s palsy. These differences probably derive from the asymmetrical compensation mechanisms employed by the bilateral hemispheres. The severity of the disease showed different associations with altered FC in some brain regions of the palsy patients. However, further study is needed to reveal the exact mechanisms underlying functional reintegration following Bell’s palsy.
Acknowledgements
Funding: This study was supported by the grants from The National Key Research and Development Program of China (No. 2016YFC0100105, 2016YFC1307001, and 2016YFC0103003), and National Natural Science Foundation of China (NSFC) (No. 81571641, 81628008, and 81771824).
Footnote
Conflicts of Interest: The authors have no conflicts of interest to declare.
Ethical Statement: The study was approved by The Ethics Committee of our hospital and written informed consent was obtained from all patients.
References
- Basić-Kes V, Dobrota VD, Cesarik M, Matovina LZ, Madzar Z, Zavoreo I, Demarin V. Peripheral facial weakness (Bell's palsy). Acta Clin Croat 2013;52:195-202. [PubMed]
- Holland NJ, Weiner GM. Recent developments in Bell's palsy. BMJ 2004;329:553-7. [Crossref] [PubMed]
- Spencer CR, Irving RM. Causes and management of facial nerve palsy. Br J Hosp Med (Lond) 2016;77:686-91. [Crossref] [PubMed]
- Hussain A, Nduka C, Moth P, Malhotra R. Bell's facial nerve palsy in pregnancy: a clinical review. J Obstet Gynaecol 2017;37:409-15. [Crossref] [PubMed]
- Portelinha J, Passarinho MP, Costa JM. Neuro-ophthalmological approach to facial nerve palsy. Saudi J Ophthalmol 2015;29:39-47. [Crossref] [PubMed]
- Gupta S, Mends F, Hagiwara M, Fatterpekar G, Roehm PC. Imaging the facial nerve: a contemporary review. Radiol Res Pract 2013;2013:248039. [Crossref] [PubMed]
- Zeng Y, Zhu J, Wang J, Parasuraman P, Busi S, Nauli SM, Wáng YXJ, Pala R, Liu G. Functional probes for cardiovascular molecular imaging. Quant Imaging Med Surg 2018;8:838-52. [Crossref] [PubMed]
- Hillman EM. Coupling mechanism and significance of the BOLD signal: a status report. Annu Rev Neurosci 2014;37:161-81. [Crossref] [PubMed]
- Fovet T, Jardri R, Linden D. Current Issues in the Use of fMRI-Based Neurofeedback to Relieve Psychiatric Symptoms. Curr Pharm Des 2015;21:3384-94. [Crossref] [PubMed]
- Conklin CJ, Faro SH, Mohamed FB. Technical considerations for functional magnetic resonance imaging analysis. Neuroimaging Clin N Am 2014;24:695-704. [Crossref] [PubMed]
- Ioannidis S, Roseman H, Chan K, Duckett S, Mizoguchi R. Posterior cortical atrophy: the value of neuroimaging in assessing memory loss. Quant Imaging Med Surg 2017;7:736-9. [Crossref] [PubMed]
- Bitter T, Sorger B, Hesselmann V, Krug B, Lackner K, Guntinas-Lichius O. Cortical representation sites of mimic movements after facial nerve reconstruction: a functional magnetic resonance imaging study. Laryngoscope 2011;121:699-706. [Crossref] [PubMed]
- Rijntjes M, Tegenthoff M, Liepert J, Leonhardt G, Kotterba S, Müller S, Kiebel S, Malin JP, Diener HC, Weiller C. Cortical reorganization in patients with facial palsy. Ann Neurol 1997;41:621-30. [Crossref] [PubMed]
- Klingner CM, Volk GF, Maertin A, Brodoehl S, Burmeister HP, Guntinas-Lichius O, Witte OW. Cortical reorganization in Bell’s palsy. Restor Neurol Neurosci 2011;29:203-14. [PubMed]
- Klingner CM, Volk GF, Brodoehl S, Burmeister HP, Witte OW, Guntinas-Lichius O. Time course of cortical plasticity after facial nerve palsy-a single case study. Neurorehabil Neural Repair 2012;26:197-203. [Crossref] [PubMed]
- Smit A, van der Geest J, Metselaar M, van der Lugt A, VanderWerf F, De Zeeuw C. Long-term changes in cerebellar activation during functional recovery from transient peripheral motor paralysis. Exp Neurol 2010;226:33-9. [Crossref] [PubMed]
- Buendia J, Loayza FR, Luis EO, Celorrio M, Pastor MA, Hontanilla B. Functional and anatomical basis for brain plasticity in facial palsy rehabilitation using the masseteric nerve. J Plast Reconstr Aesthet Surg 2016;69:417-26. [Crossref] [PubMed]
- Barkhof F, Haller S, Rombouts SA. Resting-state Functional MR imaging: A New Window to the Brain. Radiology 2014;272:29-49. [Crossref] [PubMed]
- Zacà D, Agarwal S, Gujar SK, Sair HI, Pillai JJ. Special considerations/technical limitations of blood-oxygen-level-dependent functional magnetic resonance imaging. Neuroimaging Clin N Am 2014;24:705-15. [Crossref] [PubMed]
- Jing B, Liu CH, Ma X, Yan HG, Zhuo ZZ, Zhang Y, Wang SH, Li HY, Wang CY. Difference in amplitude of low-frequency fluctuation between currently depressed and remitted females with major depressive disorder. Brain Res 2013;1540:74-83. [Crossref] [PubMed]
- Zou QH, Zhu CZ, Yang Y, Zuo XN, Long XY, Cao QJ. An improved approach to detection of amplitude of low-frequency fluctuation (ALFF) for resting-state fMRI: fractional ALFF. J Neurosci Methods 2008;172:137-41. [Crossref] [PubMed]
- He X, Zhu Y, Li C, Park K, Mohamed AZ, Wu H, Xu C, Zhang W, Wang L, Yang J, Qiu B. Acupuncture-induced changes in functional connectivity of the primary somatosensory cortex varied with pathological stages of Bell's palsy. Neuroreport 2014;25:1162-8. [Crossref] [PubMed]
- Song T, Han X, Du L, Che J, Liu J, Shi S, Fu C, Gao W, Lu J, Ma G. The role of neuroimaging in the diagnosis and treatment of depressive disorder: a recent review. Curr Pharm Des 2018;24:2515-23. [Crossref] [PubMed]
- Hu S, Wu Y, Li C, Park K, Lu G, Mohamed AZ, Wu H, Xu C, Zhang W, Wang L, Yang J, Qiu B. Increasing functional connectivity of the anterior cingulate cortex during the course of recovery from Bell's palsy. Neuroreport 2015;26:6-12. [Crossref] [PubMed]
- Mohamed AZ, Li C, Lee J. Facial Bell’s palsy affects default mode network connectivity. Chin J Magn Reson Imaging 2014;5:408-15.
- Kayhan FT, Zurakowski D, Rauch SD. Toronto Facial Grading System: interobserver reliability. Otolaryngol Head Neck Surg 2000;122:212-5. [Crossref] [PubMed]
- Yan CG, Wang XD, Zuo XN, Zang YF. DPABI: Data Processing and Analysis for (Resting-State) Brain Imaging. Neuroinformatics 2016;14:339-51. [Crossref] [PubMed]
- Qi R, Chang L, Ke J, Xu Q, Zhong J, Wang F, Zhang LJ, Lu GM. Intrinsic brain abnormalities in irritable bowel syndrome and effect of anxiety and depression. Brain Imaging Behavior 2016;10:1127-34. [Crossref] [PubMed]
- Song W, Cao Z, Lang C, Dai M, Xuan L, Lv K, Cui F, Jorgenson K, Xu M, Kong J. Disrupted functional connectivity of striatal sub-regions in Bell’s palsy patients. Neuroimage Clin 2017;14:122-9. [Crossref] [PubMed]
- Han X, Li H, Wang X, Zhu Y, Song T, Du L, Sun S, Guo R, Liu J, Shi S, Fu C, Gao W, Zhang L, Ma G. Altered Brain Fraction Amplitude of Low Frequency Fluctuation at Resting State in Patients With Early Left and Right Bell’s Palsy: Do They Have Differences? Front Neurosci 2018;12:797. [Crossref] [PubMed]
- Schaefer HS, Putnam KM, Benca RM, Davidson RJ. Event-related functional magnetic resonance imaging measures of neural activity to positive social stimuli in pre- and post-treatment depression. Biol Psychiatry 2006;60:974-86. [Crossref] [PubMed]
- Drevets WC, Savitz J, Trimble M. The subgenual anterior cingulate cortex in mood disorders. CNS Spectr 2008;13:663-81. [Crossref] [PubMed]
- Bigler ED, Mortensen S, Neeley ES, Ozonoff S, Krasny L, Johnson M, Lu J, Provencal SL, McMahon W, Lainhart JE. Superior temporal gyrus, language function, and autism. Dev Neuropsychol 2007;31:217-38. [Crossref] [PubMed]
- Jou RJ, Minshew NJ, Keshavan MS, Vitale MP, Hardan AY. Enlarged right superior temporal gyrus in children and adolescents with autism. Brain Res 2010;1360:205-12. [Crossref] [PubMed]
- Jiji S, Smitha KA, Gupta AK, Pillai VP, Jayasree RS. Segmentation and volumetric analysis of the caudate nucleus in Alzheimer's disease. Eur J Radiol 2013;82:1525-30. [Crossref] [PubMed]
- Goldberg II, Harel M, Malach R. When the brain loses its self: prefrontal inactivation during sensorimotor processing. Neuron 2006;50:329-39. [Crossref] [PubMed]
- Liu JP, Xu CS, Qi LU, Chuan-Fu LI, Yang J. Cerebral regional homogeneity of peripheral facial paralysis treated by acupuncture: a resting-state fmri study. Chin J Magn Reson Imaging 2014;5:430-5.
- Ahrens A, Skarada D, Wallace M, Cheung JY, Neely JG. Rapid simultaneous comparison system for subjective grading scales for facial paralysis. Am J Otol 1999;20:667-71. [PubMed]
- Grefkes C, Nowak DA, Wang LE, Dafotakis M, Eickhoff SB, Fink GR. Modulating cortical connectivity in stroke patients by rTMS assessed with fMRI and dynamic causal modeling. Neuroimage 2010;50:233-42. [Crossref] [PubMed]
- Hartwigsen G, Baumgaertner A, Price CJ, Koehnke M, Ulmer S, Siebner HR. Phonological decisions require both the left and right supramarginal gyri. Proc Natl Acad Sci USA 2010;107:16494-9. [Crossref] [PubMed]
- Klingner CM, Volk GF, Brodoehl S, Witte OW, Guntinas-Lichius O. The effects of deefferentation without deafferentation on functional connectivity in patients with facial palsy. Neuroimage Clin 2014;6:26-31. [Crossref] [PubMed]
- Lee J, Yang J, Li C, Yuan A, Wu H, Wang A, Xue Q, Wang T, Wang L, Gao T. Cortical Reorganization in Patients Recovered from Bell's Palsy: An Orofacial and Finger Movements Task-State fMRI Study. Neural Plast 2016;2016:8231726. [Crossref] [PubMed]
- Li C, Yang J, Sun J, Xu C, Zhu Y, Lu Q, Yuan A, Zhu Y, Li L, Zhang W, Liu J, Huang J, Chen D, Wang L, Qin W, Tian J. Brain responses to acupuncture are probably dependent on the brain functional status. Evid Based Complement Alternat Med 2013;2013:175278. [PubMed]