Quantitative MRI techniques of cartilage composition
Introduction
Osteoarthritis (OA) continues to place a large burden on society, most notably in countries with aging populations and rising rates of obesity (1,2). By 2030, 25% of the United States adult population is expected to be diagnosed with arthritis (3). As the number of patients increases, the total medical and productivity costs of the disease will also increase (4). Current research aims to prevent or reverse the effects of OA, so as to limit the individual and societal burden.
A critical aspect of this effort is the detection and monitoring of disease progression, particularly as it relates to articular cartilage. Pain scores and radiography provide some objective clinical measures, but have demonstrated only weak correlation with actual disease severity and clinical symptoms (5,6).
Magnetic resonance imaging (MRI) has emerged as a useful tool for clinicians and scientists to assess the health of cartilage and other soft tissues. Conventional MRI provides sufficient tissue contrast to detect morphological changes in cartilage where radiography cannot (7). Using these techniques, semi-quantitative scoring systems have provided a means to measure and track progression of OA (8). However, changes in cartilage physiology prior to morphological changes cannot be visualized or measured with conventional MRI (9). Detecting these more subtle changes is a priority due to the importance of detecting and managing disease at its earliest stages.
More advanced MRI techniques offer quantitative assessment of the biochemical composition of cartilage. These measures have a great potential to be used as biomarkers to track cartilage quality in clinical trials and in the study of OA pathogenesis. This article reviews the most common quantitative MR techniques and discusses the technical considerations, biochemical correlates, and advantages and disadvantages of each. Techniques discussed include delayed gadolinium enhanced MRI of cartilage (dGEMRIC), T2 mapping, T1rho mapping, sodium MRI, and a few of the more novel quantitative methods. The paper concludes with a conversation of how the field of quantitative MRI of cartilage has contributed to our understanding of two important clinical scenarios.
Physiology of cartilage
Healthy articular cartilage is optimized to reduce friction and distribute weight evenly throughout the joint. It is hypocellular, with only 4% of its wet weight consisting of chondrocytes (10). The main components of articular cartilage are water (65% to 85% of weight) and the extracellular matrix composed of type II collagen (15-20% of weight) and proteoglycans (PGs) (3-10% of weight) (11). The protein cores of PGs are lined by covalent attachments to glycosaminoglycans (GAGs), which confer negative charge due to the abundance of carboxyl and sulfate groups. This property fixes PGs to the extracellular matrix and attracts cations, such as sodium, which then draw water into the tissue to generate the swelling pressure of cartilage (10).
The biophysical structure of cartilage varies spatially between the bone interface and the articular surface to optimize different physiological characteristics (11). Several distinct layers describe this spatial variation according to depth from the articular surface (Figure 1A). The lamina splendens at the most superficial aspect of the articular surface is composed of a smooth, dense network of collagen fibers that work with synovial fluid to decrease friction and shear stress (12). Beneath this the tangential, or superficial, zone is characterized by collagen fibrils oriented parallel to the articular surface. Moving deeper, collagen orientation becomes anisotropic in the transitional zone (13) as it progresses into the radial zone, where fibrils are oriented perpendicular to the bone surface (14). Finally at the tidemark, collagen fibrils anchor cartilage to the subchondral bone.
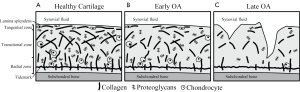
The layers described above each confer resistance to different kinds of stresses, so variation is observed throughout the joint depending on the loads and stresses applied to particular regions of cartilage. For instance, weight-bearing regions must resist strong compressive forces and therefore demonstrate thicker radial zones with greater uniformity in collagen orientation (15). Regions around the periphery of the joint are optimized to resist strong shear forces, so the transitional zone is thicker and collagen fibers within this zone are oriented in line with strongest forces (16).
In early OA, this cartilage microstructure breaks down and the tissue begins to lose functional capacity. Indications of these early changes include decreased organization of the collagen matrix, decreased fixed charge density (FCD) due to loss of PGs, and increased water content (Figure 1B) (17). These changes compromise the ability of cartilage to resist stress, resulting in further strain on the collagen matrix and leaving cartilage prone to more advanced morphological degenerative changes that conventional MRI techniques are sensitive to (Figure 1C) (18).
Quantitative MRI has demonstrated the ability to detect these changes prior to the morphological degradation of cartilage that follows as OA progresses. Thus, these novel techniques provide numeric measures that correlate with various physiological properties of cartilage and can be used to detect disease earlier than conventional imaging methods.
Quantitative MRI of cartilage
Delayed gadolinium enhanced MRI of cartilage (dGEMRIC)
dGEMRIC utilizes the FCD within cartilage to indirectly measure GAG content. The contrast agent Gd(DTPA)2- is typically injected intravenously and distributed throughout the joint during exercise prior to scanning. Within cartilage, the contrast agent experiences a repulsive force from the negatively charged GAGs and accumulates accordingly in an inverse relationship with GAG content (19). Gd(DTPA)2- is also highly paramagnetic and promotes relaxation of nearby protons following a radiofrequency (RF) pulse. This results in a reduced T1 relaxation time in regions with high levels of contrast agent. Thus, T1 relaxation is used as the quantitative outcome measure for dGEMRIC, with lower T1 indicating reduced GAG content compared to healthy cartilage (Figure 2B).
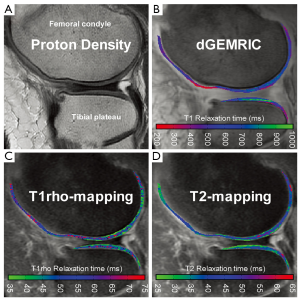
There has been some variation in the contrast administration and imaging protocol for dGEMRIC. Usually, 0.2 mmol/kg Gd(DTPA)2- is injected intravenously (20) and given time to distribute to the joint during exercise. However, alternative doses [0.1, 0.3 mmol/kg Gd(DTPA)2-] have been used (20,21) and injection is sometimes performed intra-articularly for hip imaging (22,23). The optimal delay time between injection of contrast and image acquisition also differs between joints (21). Several MRI pulse sequences can be used for dGEMRIC, most of which utilize multiple acquisitions with variable flip angles (24) or variable inversion times (25). These methods can be executed with gradient echo, spin echo, and look locker type techniques (25-27). Any of these techniques can yield T1 maps that can supply the dGEMRIC index for cartilage by averaging T1 relaxation times.
Correlation of dGEMRIC with GAG content has been validated through in vitro experiments (19,28) and has begun to be validated in vivo (29). Due to its robustness and its ability to be used in longitudinal research (30-32), dGEMRIC has been successfully applied to a wide range of clinical studies. These include tracking cartilage change after anterior cruciate ligament tear (33), performing regional analysis of patients with femoroacetabular impingement (34), and measuring damage to finger cartilage in rheumatoid arthritis (35).
While dGEMRIC remains the most established imaging tool for indirect measurement of GAG content in vivo, there are several limitations that must be considered. First, administration of contrast agent adds an invasive and potentially risky element to the protocol that is not present in other quantitative MR methods. Additionally, the delay required for the contrast to distribute throughout the joint makes for a long examination time.
T2 mapping
T2 relaxation time relates to the speed by which nuclei lose phase coherence following excitation (36). This loss of coherence results in an exponential decay of transverse magnetization and NMR signal. The rate of this decay is largely influenced by the presence of free water molecules, which slow down the loss of transverse magnetization.
Due to this relationship between T2 relaxation and free water, T2 mapping is a commonly used tool for measuring water content in cartilage (37). This method allows for the indirect assessment of collagen content and orientation, which are important indicators for early OA (38). The collagen matrix of healthy cartilage traps and immobilizes water protons, so signal intensity on T2-weighted images is low. In the earliest stages of OA, the matrix begins to break down and becomes more permeable to water, causing an elevation in T2 relaxation times (Figure 2D) (39).
T2 mapping typically involves imaging with multiple spin echo sequences at different echo times (37). 2D sequences suffer from long acquisition times and have begun to be replaced by several 3D sequences that capture the whole joint in shorter time (40-43). An additional 3D sequence known as the Double Echo Steady State (3D-DESS) allows for simultaneous estimation of T2 and apparent diffusion coefficient (ADC) values in cartilage (44,45).
The sensitivity of T2 mapping to detect changes in collagen content and orientation has been validated both in vitro (46,47) and in vivo (39,48,49). While this relationship between T2 and collagen is well-established, new evidence suggests that T2 may also be sensitive to proteoglycan content (50-52), indicating that negatively charged GAGs influence the interaction of water protons. Regardless of the precise biochemical correlate, T2 mapping has proven useful in many clinical studies. To provide a few examples, it has been used to track cartilage quality in the hips of patients with congenital hip dysplasia (53), in the knee after ACL reconstruction (54), and in the ankle after cartilage repair surgery (55).
T2 mapping is a powerful tool because it provides information regarding cartilage health without the need for contrast. However, one drawback that must be understood is the susceptibility of T2 relaxation to the magic angle effect (56). Collagen fibers at certain orientations to the B0 field influence the estimation of T2 relaxation and render T2 mapping inaccurate for these regions of cartilage. Additionally, depletion of proteoglycans is believed to occur prior to degradation of the collagen matrix in OA progression (57), so T2 mapping may not detect changes as early as techniques sensitive to GAG content, such as dGEMRIC or T1rho.
T1rho mapping
T1rho relaxation, or relaxation in the rotating frame, refers to the dissipation of energy by protons under the constant influence of a weak RF, or spin lock, pulse in the transverse plane (58). This pulse locks protons in phase to sequester T2 relaxation, and protons instead relax with the time constant T1rho.
The relaxation behavior of water in close proximity to large macromolecules (i.e., PGs) is affected by the presence of the spin lock pulse. These water protons dissipate energy faster than free water not associated with macromolecules. Due to these properties of T1rho relaxation, this quantitative measure is believed to be inversely correlated with PG or GAG content, providing an indication of early OA (Figure 2C) (59).
T1rho mapping can be accomplished with spin echo or gradient echo type pulse sequences.
The inverse relationship between T1rho relaxation time and PG/GAG content has been validated in vitro with bovine cartilage (60,61) and ex vivo with human cartilage specimens (50,62), which demonstrated a moderate to good correlation. More recent research attempting to validate this relationship in vivo has offered mixed results with weaker correlations than in vitro and ex vivo studies (51,52,63). Still, T1rho mapping has been used in a number of clinical research studies to track cartilage degradation, including patients with ACL reconstruction (64) and cartilage repair surgeries (65,66). Studies of healthy cartilage indicate that T1rho increases with age (67) and to decreases immediately following running and jumping tasks (68,69).
The major advantage of T1rho is it allows for noninvasive measurement of GAG content. One notable drawback of this method is the relatively high specific absorption rate (SAR) and potential tissue heating that results from the RF power during the spin lock preparation pulse (70).
Sodium MRI
Sodium MRI provides an additional technique for quantifying the biochemical composition of cartilage. As discussed above, sodium cations (23Na) are attracted to negatively charged GAGs and are therefore distributed in accordance with GAG content. Similar to 1H, 23Na generates an MR signal (71) that can be used to indirectly assess GAG distribution.
The ability of sodium MRI to detect differences in GAG concentration has been validated in vitro (72,73).
Numerous limitations of sodium MRI make it a less popular technique for tracking OA progression in clinical research. It is more difficult to generate an MR signal with sodium because 23Na ions exist at much lower concentrations than 1H ions within the body. In general, sufficient signal-to-noise ratio can only be achieved at higher magnetic field strengths, with special transmit and receive coils, and with long imaging times (74).
Ultrashort TE, gagCEST, and diffusion-weighted imaging (DWI)
Several other MR techniques exist that show promise in providing reliable quantitative analysis of cartilage composition. These are more novel than the aforementioned techniques and have not yet undergone rigorous validation experiments. Still, these will be of great importance looking to the future in cartilage imaging, as they likely provide new biochemical correlates and may prove more sensitive to early disease progression.
Tissues with short intrinsic relaxation times cannot be adequately assessed with the aforementioned techniques because they do not generate enough signal with MRI. These tissues include menisci, tendons, ligaments, entheses, and deep radial and calcified layers of cartilage, which all have T2* relaxation times below 5 ms (75). Ultrashort TE (UTE) pulse sequences enable T2- and T2* weighted imaging of these tissues by using an extremely short echo time (TE). T1 and T1rho measurements may also be obtained from deep cartilage layers with UTE (76).
UTE has yet to be applied in clinical research, and its correlation with aspects of cartilage composition still must be validated. A repeatability study of T2* mapping with UTE reported positive results in vivo with healthy volunteers (77). Further application of this technique to the deep radial and calcified layers of cartilage may provide critical information regarding OA pathogenesis and progression. Still, it is important to note that UTE requires lengthy scan times and will likely require development of specialized MR equipment to overcome technical challenges affecting image quality (78).
gagCEST is another novel quantitative MR technique that takes advantage of a principle known as magnetization transfer contrast (MTC) (79). MTC involves an off-resonance preparatory pulse that selectively excites and saturates immobile protons bound to macromolecules. Some of this induced transverse magnetization transfers to nearby free water protons, resulting in faster dephasing and reduced signal in free water. This creates contrast between regions with variable rates of magnetization transfer. Chemical exchange saturation transfer (CEST) is a similar technique that saturates exchangeable protons that move from macromolecules to free water, rather than transferring magnetization to free water. In articular cartilage, hydroxyl residues on GAGs are selectively excited to provide contrast between regions of high and low GAG content—gagCEST (80). This provides a direct measurement of GAG content, expressed as a magnetization transfer asymmetry value/percentage. Regions of low GAG content have low magnetization transfer and low asymmetry value.
The sensitivity of gagCEST to GAG content has been validated in vitro by comparison to sodium MRI of both healthy and diseased cartilage (81). A recent application to clinical research includes tracking change after cartilage repair with osteochondral transplantation in the knee (82). While gagCEST will continue to be applied to clinical research, several drawbacks of this technique should be understood. First, imaging at 3T is difficult due to low SNR and susceptibility of the technique to B0 inhomogeneities (83). Additionally, data analysis necessitates sophisticated post-processing tools.
Diffusion-weighted imaging (DWI) has recently provided yet another quantitative technique for measuring cartilage composition. This technique measures the translational motion of extracellular water molecules by applying diffusion-sensitizing gradients that cause mobile water protons to lose phase coherence and MR signal (84). The outcome measure for DWI is called the apparent diffusion coefficient (ADC), with higher ADC values indicating more translational motion of protons. In cartilage, this movement of free water is dependent upon the quality and orientation of collagen fibers. In cartilage, elevated ADC is believed to result from degradation of the collagen matrix, thus indicating early degeneration of cartilage (85).
As mentioned earlier, the Double Echo Steady State (DESS) is a recently developed sequence that allows for the estimation of both T2 and ADC (44). DWI has been used in a handful of clinical studies to track cartilage composition following repair surgery (55,86,87). Still, applications of DWI to cartilage imaging have proven challenging due to technical considerations for cartilage imaging, and no biochemical correlation has yet been verified.
Quantitative MRI and clinical research
Each of the above techniques has contributed to the growing body of research on OA, cartilage degradation, and treatment of focal cartilage lesions. Some of the successful clinical applications of each have briefly been mentioned, but it is also important to step back and see how the field of quantitative MRI has worked to provide knowledge regarding specific patient groups and pathologies. Two examples that are representative of this work include the growing body of research in patients with anterior cruciate ligament (ACL) tear in the knee and patients with femoroacetabular impingement (FAI) in the hip. Both of these patient groups are believed to be predisposed to early development of OA, making them ideal subjects for research of cartilage biochemistry.
ACL tear patients
Acute ACL rupture is a common traumatic injury in the active population and many of these patients develop OA in the knee (88). In a recent report on 121 ACL rupture patients five years following injury, 12% demonstrated radiographic tibiofemoral OA and 19% demonstrated radiographic patellofemoral OA (89). Ten years following ACL rupture, up to 38% of patients have presented with radiographic OA (90). These relatively high rates of OA motivate research in early detection of cartilage degradation and quantitative MRI provides a promising route to accomplish this. Even within weeks of an ACL rupture, quantitative MRI has been able to demonstrate changes in the biochemical composition of cartilage (Figure 3). These effects, along with potential recovery and longer term progression of OA, are of interest to clinicians monitoring cartilage health.
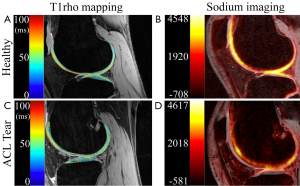
Following ACL rupture, studies have revealed elevated T1rho relaxation times in regions near bone marrow edema-like lesions (91,92), indicating rapid biochemical changes to cartilage near the site of trauma that persist up to a year following injury (93).
Recently, a study applied dGEMRIC, T2, and T1rho mapping to ACL rupture patients shortly after injury (94). Results revealed elevated T1rho and T2 relaxation times, but no focal GAG loss detected by dGEMRIC. These data suggest the traumatic event may cause an influx of water into cartilage but cause no substantial change to the supporting GAGs. This demonstrates how applications of multiple quantitative techniques can provide novel information regarding OA progression that cannot be inferred with results from a single technique.
Longitudinal studies have revealed these quantitative changes don’t necessarily recover up to one year after the initial traumatic event (95). This suggests that biochemical damage induced by ACL rupture persists, and that progression towards OA begins soon after the injury.
The cumulative results from quantitative MRI experiments have revealed much about cartilage damage following ACL tear. These methods will continue to be applied to track the success of clinical decisions and to supply further information regarding pathogenesis of OA in the knee.
Femoroacetabular impingement (FAI)
FAI is a condition caused by bone abnormalities in the hip that lead to excess friction between the femoral head, neck, and acetabular rim. Two different mechanisms of impingement are associated with FAI (cam and pincer impingement), and both are believed to be responsible for different distributions of stress around the joint. Cam impingement is caused by a lesion on the head/neck junction of the femur that causes the femoral head to be non-spherical (96). During flexion, this eccentric lesion is forced into the anterior rim of the acetabulum, compressing acetabular cartilage at its junction with the labrum (97). This stress can cause a separation of articular cartilage from the labrum and eventually lead to hip OA (98). Pincer impingement results from an overly-deep acetabulum with excessive coverage around the femoral head. Such over-coverage causes circumferential abutment between the neck of the femur and the acetabular rim, commonly resulting in compression of the labrum and increased stress to nearby acetabular cartilage around the periphery of the joint (97). While FAI may present as a single mechanism of impingement, most cases demonstrate characteristics of both cam and pincer impingement, referred to as mixed impingement (99).
It is important to note several challenges specific to imaging of hip cartilage. It is difficult to get adequate MR signal due the depth of the hip joint within the body, and relatively high spatial resolution is required to distinguish the layers of femoral and acetabular cartilage since they are only 1-3 mm thick (100). Additionally, the strong curvature of the articular surface renders hip cartilage prone magic angle effects in regions with certain orientation to B0 (101). Both in-plane and out-of-plane partial volume effects resulting from this curvature also make hip imaging challenging.
dGEMRIC has been one of the leading quantitative techniques for hip cartilage imaging. In a study by Bittersohl et al., dGEMRIC was applied to 26 symptomatic FAI patients and ten asymptomatic volunteers (102). Comparison between groups revealed significantly lower T1 relaxation times in FAI cartilage, suggesting a marked reduction in GAG content. Additionally, regional analysis of purely cam- and pincer-types revealed concentration of GAG depletion in the anterior-superior region for cam-type and more circumferential GAG depletion for pincer-type. A later study by the same group compared dGEMRIC imaging to intra-operative findings and found reduced T1 values in damaged cartilage (103). Still, T1 values were only weakly correlated with intra-operative determination of healthy versus damaged cartilage. Overall, applications of dGEMRIC suggest that GAG depletion occurs in patients with FAI, and that these effects may vary regionally according to the mechanism of impingement.
T2* mapping has also made a contribution to research on cartilage damage with FAI. A recent study demonstrated a significant reduction in T2* relaxation time for morphologically normal-appearing cartilage in FAI patients compared to cartilage of asymptomatic volunteers (104). This suggests that early biochemical changes to cartilage are occurring with impingement, and that novel quantitative MR techniques may be sensitive to such changes.
T1rho and T2 mapping also continue to be applied to image cartilage in the hip (Figure 4), and provide a non-invasive measure of cartilage composition.
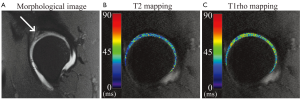
One major difficulty in validating quantitative MRI is that there is no highly-sensitive reference standard with which to compare. As demonstrated by the studies above, quantitative outcomes were compared with morphological findings from conventional MRI, with intra-operative findings, or with data from healthy subjects. None of these are sensitive to early biochemical degradation of cartilage, so it is difficult to firmly establish that quantitative differences are due to changes such as PG loss, collagen matrix decomposition, and increased water content. Future validation with histological grading of cartilage will serve as a powerful test of quantitative techniques in hip cartilage.
Conclusions
The field of quantitative MRI is rapidly evolving, and while more established techniques are applied to clinical research, new techniques continue to be tested as methods for capturing cartilage composition. Each offers unique advantages and disadvantages for cartilage imaging. Looking to the future, determination of biochemical correlates for each quantitative technique will be essential in developing and tracking interventions to prevent OA progression. Finally, while research often focuses on developing and validating single techniques, successful applications to clinical research may come best when combining multiple quantitative methods.
Acknowledgements
Disclosure: No financial support from federal or industry sources have been received for the work reported in the manuscript. Garry E. Gold receives research support from GE Healthcare., and is a consultant for Zimmer, Boston Scientific and Isto Inc. All other authors report no financial interests that could create a potential conflict of interest with regard to the work.
References
- Buckwalter JA, Saltzman C, Brown T. The impact of osteoarthritis: implications for research. Clin Orthop Relat Res 2004;(427 Suppl):S6-15. [PubMed]
- Woolf AD, Pfleger B. Burden of major musculoskeletal conditions. Bull World Health Organ 2003;81:646-56. [PubMed]
- Hootman JM, Helmick CG. Projections of US prevalence of arthritis and associated activity limitations. Arthritis Rheum 2006;54:226-9. [PubMed]
- Hermans J, Koopmanschap MA, Bierma-Zeinstra SM, et al. Productivity costs and medical costs among working patients with knee osteoarthritis. Arthritis Care Res (Hoboken) 2012;64:853-61. [PubMed]
- Finan PH, Buenaver LF, Bounds SC, et al. Discordance between pain and radiographic severity in knee osteoarthritis: findings from quantitative sensory testing of central sensitization. Arthritis Rheum 2013;65:363-72. [PubMed]
- Kijowski R, Blankenbaker DG, Stanton PT, et al. Radiographic findings of osteoarthritis versus arthroscopic findings of articular cartilage degeneration in the tibiofemoral joint. Radiology 2006;239:818-24. [PubMed]
- Koster IM, Oei EH, Hensen JH, et al. Predictive factors for new onset or progression of knee osteoarthritis one year after trauma: MRI follow-up in general practice. Eur Radiol 2011;21:1509-16. [PubMed]
- Guermazi A, Roemer FW, Haugen IK, et al. MRI-based semiquantitative scoring of joint pathology in osteoarthritis. Nat Rev Rheumatol 2013;9:236-51. [PubMed]
- Zhang M, Min Z, Rana N, et al. Accuracy of magnetic resonance imaging in grading knee chondral defects. Arthroscopy 2013;29:349-56. [PubMed]
- Maroudas A, Bayliss MT, Venn MF. Further studies on the composition of human femoral head cartilage. Ann Rheum Dis 1980;39:514-23. [PubMed]
- Venn M, Maroudas A. Chemical composition and swelling of normal and osteoarthrotic femoral head cartilage. I. Chemical composition. Ann Rheum Dis 1977;36:121-9. [PubMed]
- Jay GD, Torres JR, Warman ML, et al. The role of lubricin in the mechanical behavior of synovial fluid. Proc Natl Acad Sci U S A 2007;104:6194-9. [PubMed]
- Clark JM. The organization of collagen in cryofractured rabbit articular cartilage: a scanning electron microscopic study. J Orthop Res 1985;3:17-29. [PubMed]
- Clark JM. Variation of collagen fiber alignment in a joint surface: a scanning electron microscope study of the tibial plateau in dog, rabbit, and man. J Orthop Res 1991;9:246-57. [PubMed]
- Moger CJ, Barrett R, Bleuet P, et al. Regional variations of collagen orientation in normal and diseased articular cartilage and subchondral bone determined using small angle X-ray scattering (SAXS). Osteoarthritis Cartilage 2007;15:682-7. [PubMed]
- Thompson AM, Stockwell RA. An ultrastructural study of the marginal transitional zone in the rabbit knee joint. J Anat 1983;136:701-13. [PubMed]
- Buckwalter JA, Mankin HJ. Articular cartilage: degeneration and osteoarthritis, repair, regeneration, and transplantation. Instr Course Lect 1998;47:487-504. [PubMed]
- Dijkgraaf LC, de Bont LG, Boering G, et al. The structure, biochemistry, and metabolism of osteoarthritic cartilage: a review of the literature. J Oral Maxillofac Surg 1995;53:1182-92. [PubMed]
- Bashir A, Gray ML, Burstein D. Gd-DTPA2- as a measure of cartilage degradation. Magn Reson Med 1996;36:665-73. [PubMed]
- Tiderius CJ, Olsson LE, de Verdier H, et al. Gd-DTPA2)-enhanced MRI of femoral knee cartilage: a dose-response study in healthy volunteers. Magn Reson Med 2001;46:1067-71. [PubMed]
- Burstein D, Velyvis J, Scott KT, et al. Protocol issues for delayed Gd(DTPA)(2-)-enhanced MRI (dGEMRIC) for clinical evaluation of articular cartilage. Magn Reson Med 2001;45:36-41. [PubMed]
- Bittersohl B, Hosalkar HS, Werlen S, et al. Intravenous versus intra-articular delayed gadolinium-enhanced magnetic resonance imaging in the hip joint: a comparative analysis. Invest Radiol 2010;45:538-42. [PubMed]
- Boesen M, Jensen KE, Qvistgaard E, et al. Delayed gadolinium-enhanced magnetic resonance imaging (dGEMRIC) of hip joint cartilage: better cartilage delineation after intra-articular than intravenous gadolinium injection. Acta Radiol 2006;47:391-6. [PubMed]
- Trattnig S, Marlovits S, Gebetsroither S, et al. Three-dimensional delayed gadolinium-enhanced MRI of cartilage (dGEMRIC) for in vivo evaluation of reparative cartilage after matrix-associated autologous chondrocyte transplantation at 3.0T: Preliminary results. J Magn Reson Imaging 2007;26:974-82. [PubMed]
- McKenzie CA, Williams A, Prasad PV, et al. Three-dimensional delayed gadolinium-enhanced MRI of cartilage (dGEMRIC) at 1.5T and 3.0T. J Magn Reson Imaging 2006;24:928-33. [PubMed]
- Tiderius CJ, Jessel R, Kim YJ, et al. Hip dGEMRIC in asymptomatic volunteers and patients with early osteoarthritis: the influence of timing after contrast injection. Magn Reson Med 2007;57:803-5. [PubMed]
- Kimelman T, Vu A, Storey P, et al. Three-dimensional T1 mapping for dGEMRIC at 3.0 T using the Look Locker method. Invest Radiol 2006;41:198-203. [PubMed]
- Bashir A, Gray ML, Hartke J, et al. Nondestructive imaging of human cartilage glycosaminoglycan concentration by MRI. Magn Reson Med 1999;41:857-65. [PubMed]
- Watanabe A, Wada Y, Obata T, et al. Delayed gadolinium-enhanced MR to determine glycosaminoglycan concentration in reparative cartilage after autologous chondrocyte implantation: preliminary results. Radiology 2006;239:201-8. [PubMed]
- Multanen J, Rauvala E, Lammentausta E, et al. Reproducibility of imaging human knee cartilage by delayed gadolinium-enhanced MRI of cartilage (dGEMRIC) at 1.5 Tesla. Osteoarthritis Cartilage 2009;17:559-64. [PubMed]
- Siversson C, Tiderius CJ, Neuman P, et al. Repeatability of T1-quantification in dGEMRIC for three different acquisition techniques: two-dimensional inversion recovery, three-dimensional look locker, and three-dimensional variable flip angle. J Magn Reson Imaging 2010;31:1203-9. [PubMed]
- van Tiel J, Bron EE, Tiderius CJ, et al. Reproducibility of 3D delayed gadolinium enhanced MRI of cartilage (dGEMRIC) of the knee at 3.0 T in patients with early stage osteoarthritis. Eur Radiol 2013;23:496-504. [PubMed]
- Neuman P, Tjörnstrand J, Svensson J, et al. Longitudinal assessment of femoral knee cartilage quality using contrast enhanced MRI (dGEMRIC) in patients with anterior cruciate ligament injury--comparison with asymptomatic volunteers. Osteoarthritis Cartilage 2011;19:977-83. [PubMed]
- Mamisch TC, Kain MS, Bittersohl B, et al. Delayed gadolinium-enhanced magnetic resonance imaging of cartilage (dGEMRIC) in Femoacetabular impingement. J Orthop Res 2011;29:1305-11. [PubMed]
- Miese F, Buchbender C, Scherer A, et al. Molecular imaging of cartilage damage of finger joints in early rheumatoid arthritis with delayed gadolinium-enhanced magnetic resonance imaging. Arthritis Rheum 2012;64:394-9. [PubMed]
- Plewes DB, Kucharczyk W. Physics of MRI: a primer. J Magn Reson Imaging 2012;35:1038-54. [PubMed]
- Mosher TJ, Dardzinski BJ. Cartilage MRI T2 relaxation time mapping: overview and applications. Semin Musculoskelet Radiol 2004;8:355-68. [PubMed]
- David-Vaudey E, Ghosh S, Ries M, et al. T2 relaxation time measurements in osteoarthritis. Magn Reson Imaging 2004;22:673-82. [PubMed]
- Dardzinski BJ, Mosher TJ, Li S, et al. Spatial variation of T2 in human articular cartilage. Radiology 1997;205:546-50. [PubMed]
- Quaia E, Toffanin R, Guglielmi G, et al. Fast T2 mapping of the patellar articular cartilage with gradient and spin-echo magnetic resonance imaging at 1.5 T: validation and initial clinical experience in patients with osteoarthritis. Skeletal Radiol 2008;37:511-7. [PubMed]
- Van Breuseghem I, Bosmans HT, Elst LV, et al. T2 mapping of human femorotibial cartilage with turbo mixed MR imaging at 1.5 T: feasibility. Radiology 2004;233:609-14. [PubMed]
- Chen W, Takahashi A, Han ET. 3D Quantitative Imaging of T1rho and T2. In: 19th, editor. International Society for Magnetic Resonance in Medicine: Annual Meeting & Exhibition; Montréal 2011.
- Chen W, Zhang T, Han ET, et al. Joint anatomical and biochemical imaging using 3D FSE. In: 19th, editor. International Society for Magnetic Resonance in Medicine: Annual Meeting & Exhibition; Montréal 2011.
- Staroswiecki E, Granlund KL, Alley MT, et al. Simultaneous estimation of T(2) and apparent diffusion coefficient in human articular cartilage in vivo with a modified three-dimensional double echo steady state (DESS) sequence at 3 T. Magn Reson Med 2012;67:1086-96. [PubMed]
- Welsch GH, Mamisch TC, Zak L, et al. Morphological and biochemical T2 evaluation of cartilage repair tissue based on a hybrid double echo at steady state (DESS-T2d) approach. J Magn Reson Imaging 2011;34:895-903. [PubMed]
- Dunn TC, Lu Y, Jin H, et al. T2 relaxation time of cartilage at MR imaging: comparison with severity of knee osteoarthritis. Radiology 2004;232:592-8. [PubMed]
- Nissi MJ, Töyräs J, Laasanen MS, et al. Proteoglycan and collagen sensitive MRI evaluation of normal and degenerated articular cartilage. J Orthop Res 2004;22:557-64. [PubMed]
- Yao W, Qu N, Lu Z, et al. The application of T1 and T2 relaxation time and magnetization transfer ratios to the early diagnosis of patellar cartilage osteoarthritis. Skeletal Radiol 2009;38:1055-62. [PubMed]
- Smith HE, Mosher TJ, Dardzinski BJ, et al. Spatial variation in cartilage T2 of the knee. J Magn Reson Imaging 2001;14:50-5. [PubMed]
- Keenan KE, Besier TF, Pauly JM, et al. Prediction of glycosaminoglycan content in human cartilage by age, T1ρ and T2 MRI. Osteoarthritis Cartilage 2011;19:171-9. [PubMed]
- Nishioka H, Hirose J, Nakamura E, et al. T1ρ and T2 mapping reveal the in vivo extracellular matrix of articular cartilage. J Magn Reson Imaging 2012;35:147-55. [PubMed]
- Wong CS, Yan CH, Gong NJ, et al. Imaging biomarker with T1ρ and T2 mappings in osteoarthritis - in vivo human articular cartilage study. Eur J Radiol 2013;82:647-50. [PubMed]
- Nishii T, Tanaka H, Sugano N, et al. Evaluation of cartilage matrix disorders by T2 relaxation time in patients with hip dysplasia. Osteoarthritis Cartilage 2008;16:227-33. [PubMed]
- Van Ginckel A, Verdonk P, Victor J, et al. Cartilage status in relation to return to sports after anterior cruciate ligament reconstruction. Am J Sports Med 2013;41:550-9. [PubMed]
- Quirbach S, Trattnig S, Marlovits S, et al. Initial results of in vivo high-resolution morphological and biochemical cartilage imaging of patients after matrix-associated autologous chondrocyte transplantation (MACT) of the ankle. Skeletal Radiol 2009;38:751-60. [PubMed]
- Levitt M. Spin dynamics: basics of nuclear magnetic resonance. New York: Wiley;2008.
- Pearle AD, Warren RF, Rodeo SA. Basic science of articular cartilage and osteoarthritis. Clin Sports Med 2005;24:1-12. [PubMed]
- Borthakur A, Mellon E, Niyogi S, et al. Sodium and T1rho MRI for molecular and diagnostic imaging of articular cartilage. NMR Biomed 2006;19:781-821. [PubMed]
- Duvvuri U, Reddy R, Patel SD, et al. T1rho-relaxation in articular cartilage: effects of enzymatic degradation. Magn Reson Med 1997;38:863-7. [PubMed]
- Duvvuri U, Kudchodkar S, Reddy R, et al. T(1rho) relaxation can assess longitudinal proteoglycan loss from articular cartilage in vitro. Osteoarthritis Cartilage 2002;10:838-44. [PubMed]
- Regatte RR, Akella SV, Borthakur A, et al. Proton spin-lock ratio imaging for quantitation of glycosaminoglycans in articular cartilage. J Magn Reson Imaging 2003;17:114-21. [PubMed]
- Li X, Cheng J, Lin K, et al. Quantitative MRI using T1ρ and T2 in human osteoarthritic cartilage specimens: correlation with biochemical measurements and histology. Magn Reson Imaging 2011;2:324-34.
- Tsushima H, Okazaki K, Takayama Y, et al. Evaluation of cartilage degradation in arthritis using T1ρ magnetic resonance imaging mapping. Rheumatol Int 2012;32:2867-75. [PubMed]
- Theologis AA, Haughom B, Liang F, et al. Comparison of T1rho relaxation times between ACL-reconstructed knees and contralateral uninjured knees. Knee Surg Sports Traumatol Arthrosc 2013. [Epub ahead of print]. [PubMed]
- Theologis AA, Schairer WW, Carballido-Gamio J, et al. Longitudinal analysis of T1ρ and T2 quantitative MRI of knee cartilage laminar organization following microfracture surgery. Knee 2012;19:652-7. [PubMed]
- Holtzman DJ, Theologis AA, Carballido-Gamio J, et al. T(1ρ) and T(2) quantitative magnetic resonance imaging analysis of cartilage regeneration following microfracture and mosaicplasty cartilage resurfacing procedures. J Magn Reson Imaging 2010;32:914-23. [PubMed]
- Goto H, Iwama Y, Fujii M, et al. A preliminary study of the T1rho values of normal knee cartilage using 3T-MRI. Eur J Radiol 2012;81:e796-803. [PubMed]
- Subburaj K, Kumar D, Souza RB, et al. The acute effect of running on knee articular cartilage and meniscus magnetic resonance relaxation times in young healthy adults. Am J Sports Med 2012;40:2134-41. [PubMed]
- Souza RB, Fang C, Luke A, et al. Relationship between knee kinetics during jumping tasks and knee articular cartilage MRI T1rho and T2 relaxation times. Clin Biomech (Bristol, Avon) 2012;27:403-8. [PubMed]
- Pedersen DR, Klocke NF, Thedens DR, et al. Integrating carthage-specific T1rho MRI into knee clinic diagnostic imaging. Iowa Orthop J 2011;31:99-109. [PubMed]
- Granot J. Sodium imaging of human body organs and extremities in vivo. Radiology 1988;167:547-50. [PubMed]
- Insko EK, Kaufman JH, Leigh JS, et al. Sodium NMR evaluation of articular cartilage degradation. Magn Reson Med 1999;41:30-4. [PubMed]
- Borthakur A, Shapiro EM, Beers J, et al. Sensitivity of MRI to proteoglycan depletion in cartilage: comparison of sodium and proton MRI. Osteoarthritis Cartilage 2000;8:288-93. [PubMed]
- Gold GE, Hargreaves BA, Stevens KJ, Beaulieu CF. Advanced magnetic resonance imaging of articular cartilage. Orthop Clin North Am 2006;37:331-47. [PubMed]
- Du J, Takahashi AM, Chung CB. Ultrashort TE spectroscopic imaging (UTESI): application to the imaging of short T2 relaxation tissues in the musculoskeletal system. J Magn Reson Imaging 2009;29:412-21. [PubMed]
- Du J, Carl M, Bae WC, et al. Dual inversion recovery ultrashort echo time (DIR-UTE) imaging and quantification of the zone of calcified cartilage (ZCC). Osteoarthritis Cartilage 2013;21:77-85. [PubMed]
- Williams A, Qian Y, Chu CR. UTE-T2* mapping of human articular cartilage in vivo: a repeatability assessment. Osteoarthritis Cartilage 2011;19:84-8. [PubMed]
- Tyler DJ, Robson MD, Henkelman RM, et al. Magnetic resonance imaging with ultrashort TE (UTE) PULSE sequences: technical considerations. J Magn Reson Imaging 2007;25:279-89. [PubMed]
- Wolff SD, Balaban RS. Magnetization transfer contrast (MTC) and tissue water proton relaxation in vivo. Magn Reson Med 1989;10:135-44. [PubMed]
- Ling W, Regatte RR, Navon G, et al. Assessment of glycosaminoglycan concentration in vivo by chemical exchange-dependent saturation transfer (gagCEST). Proc Natl Acad Sci U S A 2008;105:2266-70. [PubMed]
- Schmitt B, Zbýn S, Stelzeneder D, et al. Cartilage quality assessment by using glycosaminoglycan chemical exchange saturation transfer and (23)Na MR imaging at 7 T. Radiology 2011;260:257-64. [PubMed]
- Krusche-Mandl I, Schmitt B, Zak L, et al. Long-term results 8 years after autologous osteochondral transplantation: 7 T gagCEST and sodium magnetic resonance imaging with morphological and clinical correlation. Osteoarthritis Cartilage 2012;20:357-63. [PubMed]
- Singh A, Haris M, Cai K, et al. Chemical exchange saturation transfer magnetic resonance imaging of human knee cartilage at 3 T and 7 T. Magn Reson Med 2012;68:588-94. [PubMed]
- Gold GE, Burstein D, Dardzinski B, et al. MRI of articular cartilage in OA: novel pulse sequences and compositional/functional markers. Osteoarthritis Cartilage 2006;14 Suppl A:A76-86.
- Mlynárik V, Sulzbacher I, Bittsanský M, et al. Investigation of apparent diffusion constant as an indicator of early degenerative disease in articular cartilage. J Magn Reson Imaging 2003;17:440-4. [PubMed]
- Friedrich KM, Mamisch TC, Plank C, et al. Diffusion-weighted imaging for the follow-up of patients after matrix-associated autologous chondrocyte transplantation. Eur J Radiol 2010;73:622-8. [PubMed]
- Apprich S, Trattnig S, Welsch GH, et al. Assessment of articular cartilage repair tissue after matrix-associated autologous chondrocyte transplantation or the microfracture technique in the ankle joint using diffusion-weighted imaging at 3 Tesla. Osteoarthritis Cartilage 2012;20:703-11. [PubMed]
- Øiestad BE, Engebretsen L, Storheim K, et al. Knee osteoarthritis after anterior cruciate ligament injury: a systematic review. Am J Sports Med 2009;37:1434-43. [PubMed]
- Frobell RB, Roos HP, Roos EM, et al. Treatment for acute anterior cruciate ligament tear: five year outcome of randomised trial. BMJ 2013;346:f232. [PubMed]
- Meuffels DE, Favejee MM, Vissers MM, et al. Ten year follow-up study comparing conservative versus operative treatment of anterior cruciate ligament ruptures. A matched-pair analysis of high level athletes. Br J Sports Med 2009;43:347-51. [PubMed]
- Bolbos RI, Ma CB, Link TM, et al. In vivo T1rho quantitative assessment of knee cartilage after anterior cruciate ligament injury using 3 Tesla magnetic resonance imaging. Invest Radiol 2008;43:782-8. [PubMed]
- Li X, Ma BC, Bolbos RI, et al. Quantitative assessment of bone marrow edema-like lesion and overlying cartilage in knees with osteoarthritis and anterior cruciate ligament tear using MR imaging and spectroscopic imaging at 3 Tesla. J Magn Reson Imaging 2008;28:453-61. [PubMed]
- Theologis AA, Kuo D, Cheng J, et al. Evaluation of bone bruises and associated cartilage in anterior cruciate ligament-injured and -reconstructed knees using quantitative t(1ρ) magnetic resonance imaging: 1-year cohort study. Arthroscopy 2011;27:65-76. [PubMed]
- Klocke NF, Amendola A, Thedens DR, et al. Comparison of T1ρ, dGEMRIC, and quantitative T2 MRI in preoperative ACL rupture patients. Acad Radiol 2013;20:99-107. [PubMed]
- Li X, Kuo D, Theologis A, et al. Cartilage in anterior cruciate ligament-reconstructed knees: MR imaging T1{rho} and T2--initial experience with 1-year follow-up. Radiology 2011;258:505-14. [PubMed]
- Beck M, Chegini S, Ferguson S, et al. Mechanism of Femoroacetabular Impingement. In: Marin-Pena O. eds. Femoroacetabular Impingement. Berlin, Heidelberg: Springer Berlin Heidelberg, 2012:9-22.
- Beck M, Kalhor M, Leunig M, et al. Hip morphology influences the pattern of damage to the acetabular cartilage: femoroacetabular impingement as a cause of early osteoarthritis of the hip. J Bone Joint Surg Br 2005;87:1012-8. [PubMed]
- Agricola R, Heijboer MP, Bierma-Zeinstra SM, et al. Cam impingement causes osteoarthritis of the hip: a nationwide prospective cohort study (CHECK). Ann Rheum Dis 2013;72:918-23. [PubMed]
- Ganz R, Leunig M, Leunig-Ganz K, et al. The etiology of osteoarthritis of the hip: an integrated mechanical concept. Clin Orthop Relat Res 2008;466:264-72. [PubMed]
- Kurrat HJ, Oberländer W. The thickness of the cartilage in the hip joint. J Anat 1978;126:145-55. [PubMed]
- Xia Y. Magic-angle effect in magnetic resonance imaging of articular cartilage: a review. Invest Radiol 2000;35:602-21. [PubMed]
- Bittersohl B, Steppacher S, Haamberg T, et al. Cartilage damage in femoroacetabular impingement (FAI): preliminary results on comparison of standard diagnostic vs delayed gadolinium-enhanced magnetic resonance imaging of cartilage (dGEMRIC). Osteoarthritis Cartilage 2009;17:1297-306. [PubMed]
- Bittersohl B, Hosalkar HS, Apprich S, et al. Comparison of pre-operative dGEMRIC imaging with intra-operative findings in femoroacetabular impingement: preliminary findings. Skeletal Radiol 2011;40:553-61. [PubMed]
- Bittersohl B, Miese FR, Hosalkar HS, et al. T2* mapping of acetabular and femoral hip joint cartilage at 3 T: a prospective controlled study. Invest Radiol 2012;47:392-7. [PubMed]