Anatomical digital twins for medical education: a stepwise guide to create perpetual multimodal three-dimensional reconstruction of digital brain specimens
Introduction
Neuroanatomy is a critical component of medical education, and understanding the morphology and interposition relationships of the different anatomical structures of the human brain is a crucial but complex aspect of the learning process, particularly for surgical training (1,2). The development and application of biomedical imaging technologies have greatly facilitated the digitization of human specimens (1,3-7). In recent years, rapid advances in complex mathematical models, image-processing techniques, and visualization tools have made it possible to display complex three-dimensional (3D) anatomical structures (1,8-14).
For decades, the study of obsolete human specimens in medical curricula has relied heavily upon traditional, nonrepeatable dissection methods. Such an approach leads to suboptimal specimen utilization, and all the inherent financial and bioethical implications (15). Even in conjunction with anatomy textbooks, it is difficult for our medical students and surgical trainees to understand the relationships between adjacent neuroanatomical structures using their imagination alone (1). Although latex injection techniques have evolved, becoming a mature and reliable technique ubiquitously available in cadaver laboratories, the intravascular injection of contrast agents containing rubber is much more suitable for the digital imaging of fresh human specimens (16-18). Nonetheless, obtaining fresh human specimens remains problematic in countries without legislation on donation of human bodies for scientific purposes, and even where this is not an issue, medical schools tend to have a limited inventory of anatomical specimens (19).
We therefore aimed to create a cost-effective and reproducible technique for image acquisition and 3D reconstruction of digital models from adult human brain specimens, even if they are not fresh (>2 years postmortem). Computed tomography (CT) scans represent the gold standard for studying bony structures such as the calvaria and skull base, whereas magnetic resonance imaging (MRI) scans offer ideal contrast for soft tissues, such as the brain and spinal cord (20,21). By endovascularly injecting a contrast agent into selected specimens, we attempted to reconstruct the vascular structure from large arteries to capillaries, from veins to venous sinuses, so that the entire vascular system would be visible on CT and MRI scans and thus ultimately be amenable to conversion into Digital Imaging and Communications in Medicine (DICOM) format. Aged specimens may have collapsed blood vessels due to blood loss, but the original model of the specimen can be restored to some degree by the vascular perfusion technique (1,22,23).
After formaldehyde fixation, image editing and 3D optimized reconstruction of aged specimens can be achieved via modern 3D reconstruction software (e.g., the open-source 3D Slicer platform; https://www.slicer.org/) for high-quality digital display (8,24). This method can be used not only for the creation of digital models but also for the reproduction of 3D printing models or mixed-reality ones, all holding the potential to be widely used in areas such as medical anatomy teaching and surgical training (14,25-27).
The combination of computerized 3D modeling techniques with gross anatomy enables the scanning of specimens before dissection to facilitate visualization of the internal structure of the model (28-30). This technique has a wide range of applications, as it allows for more intuitive and confidently performed surgical procedures (due to the “preview” imaging provided to the operator), hence it is particularly suitable for surgical training scenarios (1,13,31). Moreover, this process enhances the surgeon’s routine operational planning of any given surgical corridor by shifting from the assessment of preoperative two-dimensional (2D) images to 3D image reconstruction models adequate for virtual reality environments (28,32).
Methods
Selection and acquisition of specimens
This study was performed on four human cadaveric heads of Asian adults from individuals who donated their bodies to the Human Anatomy Teaching and Research Laboratory of Sun Yat-sen University for scientific research and education. The study was conducted in accordance with the Declaration of Helsinki (as revised in 2013). This study was conducted with the consent of the donors and their families, in accordance with articles 1, 3, and 5 of the Administrative Measures of Guangzhou Municipality on Voluntary Body Donation. The requirement for ethical approval was waived by the Ethics Committee of the Third Affiliated Hospital of Sun Yat-sen University as the individuals had willingly donated their bodies for scientific purposes. Only the age and gender of the individuals were known to the investigators.
The cadavers were stored in the specimen bank of the Human Anatomy Teaching and Research Laboratory of Sun Yat-sen University and were aged formaldehyde-fixed specimens used in the medical curriculum. We selected specimens that had been preserved in formaldehyde for more than 3 years and had not been damaged by extensive dissection. Based on the above screening criteria, we randomly selected four specimens for this experiment. Complete and clear vessels of the internal carotid artery (ICA), vertebral artery (VA), and jugular vein were visible at the base of the specimens, except for one specimen in which the VA could not be seen.
Image acquisition methods
According to our pre-experiment, we knew that the bony tissue of the skull could be preserved intact and that the brain tissues, although atrophied, still retained a certain shape due to timely formalin fixation. Therefore, the shape information could be obtained by CT or MRI scanning, but the vasculature could not be scanned due to its unfilled nature and the microcirculation between arterioles and veins no longer being patent (33). Therefore, we constructed a simple vascular perfusion model to fill the arteries and veins separately, rendering them amenable to scanning, and acquired the final scanned images for 3D reconstruction.
CT contrast agents include iodine contrast, which can highlight the vascular shape by increasing the density difference between the lesion and the neighboring tissues. Similarly, contrast agents for MRI, such as gadolinium diamine, can shorten the T1 and T2 relaxation times of protons in tissues, thus enhancing the clarity and contrast of the image for vascular visualization. It is worth mentioning that the conventional magnetic resonance (MR) angiography time-of-flight technique, which identifies and visualizes arteries based on flow velocity, would typically be used in this context, but since the dynamic flow velocity of the blood vessels of these specimens could not be restored, we used the injection of the contrast agent to achieve the purpose of vascular visualization.
For the configuration of the contrast agent, we preliminarily explored the developmental effect of different concentrations of CT and MRI contrast agent mixtures with water, and after considering the cost and quality, we chose a 1:10 solution of CT contrast agent (iodixanol, 100 mL:32 g) and a 1:10 solution of MRI contrast agent (gadolinium diamine, 15 mL:4.305 g) (Figure 1). After our preliminary study, we found that the CT contrast agent had no significant effect under MR scanning, so we performed CT perfusion scanning before MR perfusion scanning on the specimens. Since the vascular perfusion in this experiment did not involve forensic identification, the use of aqueous contrast agents was feasible, which has the advantage of easy injection and availability (21,34). After being processed by mixing with water at a ratio of 1:10, the contrast agent was a colorless liquid, and it did not appear to have damaged the specimen to the naked eye and could be used in the medical curriculum.
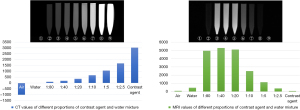
Preprocessing of specimens
First, the blood vessels in the neck were identified and dissected. A 1.5–2-cm length of each vessel was separated from the surrounding soft tissues and exposed; the lead end of the disposable intravenous catheter was cut at an angle of 45°. Considering the walls of the old vessels had lost their elasticity after immobilization, instead of using balloon fixation, we used sutures to tighten the overlap between the vessels and the catheter. We tested the tightness of this approach and tightened the exposed catheter with the remaining sutures to ensure a watertight seal and prevent accidental displacement. The ICA was first identified, and the catheter mentioned above was inserted 2–3 cm into the vessel. Next, the catheter was inserted into the same side of the vessel in the same manner, in an ICA-VA-internal jugular vein (IJV) sequence (Figure 2A). As we did not require revascularization of the external carotid artery (ECA), we clamped the ECA.
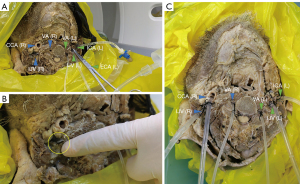
In the second step, after cannulation, the specimen vessel received a slow, continuous injection of water with the primary goal of refilling the vessel and draining it of thrombus and tissue debris. After the completion of intubation, the other end of the hose was connected to a three-way tube, which could be flexibly connected to the external pipeline and closed or opened at any time. Subsequently, a syringe was used to inject water slowly and continuously into the vessel so as not to collapse the vessel due to rapid filling and tearing, which was observed to gradually fill and dilate, with a cloudy, dark-red fluid and irregular material emerging from the other end of the vessel (Figure 2B). Over time, the outflow gradually became lighter in color while the amount of solid material decreased, indicating that the residual thrombus or tissue debris in the lumen had cleared. Since the perfusion of the venous system may affect the flushing and dilation of the arterial system, the arterial system should be flushed before the venous system to avoid unnecessary pressure changes. Therefore, we employed an ICA-VA-dominant IJV (usually on the right side)-nondominant IJV flushing sequence (32).
The primary objectives of the above-described procedure were the following: (I) to gradually fill the vessels in the cranial brain specimen without rupturing them using a continuous slow, low-pressure flow of water; (II) to facilitate the outflow of material (e.g., thrombus and tissue debris) left in the vessels to minimize the impact on the intravascular perfusion of the contrast medium on the subsequent scanning and imaging; (III) to assess the vascular system, determine the patency, and predict resistance in the arterial system through observation; and (IV) to ligate the external carotid system or the superficial branch should their blood flow enter the internal carotid system (16). With these objectives obtained, the third step was the connection to the other side of the vessel, and the preparation of the vessel for perfusion (Figure 2C) could be implemented. It is important to note that at this stage, there was residual formaldehyde solution in the specimen intracranially along with injected fluid flow due to rupture of the vessel; therefore, the specimen needed to be placed in a vertical position to allow for as much unwanted fluid as possible to drain out of the specimen. This is an important technical tip for all those who wish to apply our stepwise guide in their own anatomical laboratories.
Vascular perfusion and image scanning
After specimen pretreatment, a mixture of CT and MRI contrast agents and water was injected into the treated vessel according to the imaging principle. The method of configuration was simple, as no additional substances were required for configuration, and both contrast agents had a wide range of developing concentrations. After a short wait, the cranial brain specimens were scanned by CT and MRI, respectively, and enhanced CT and MRI DICOM images were obtained, which were then exported to the open-source 3D Slicer software platform (version 5.6.2) for 3D reconstruction and optimization (Figure 3).
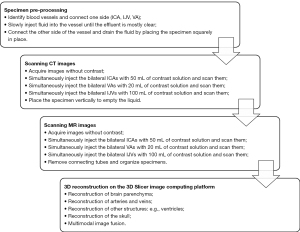
During contrast infusion, we used the protocol of simultaneous injection of contrast agents on both sides. We followed this with scanning because, unlike fresh specimens, aged specimens are likely to have internal vascular disruptions, the location of which is unknown; however, after simultaneous bilateral injection, CT scanning can locate these disruptions, thus serving as a guide for the infusion of the contrast agent during MRI scanning. Additionally, simultaneous bilateral perfusion increases intravascular pressure and may induce blood flow through the traffic vessels within the circle of Willis. Since the quality of the specimen in our study was unknown and variable (a decision which was deliberately made to increase the replicability of our results elsewhere, including in laboratories from low- and middle-income countries), the perfusion and scanning process was dynamic, and the dynamic results of the CT scan could demonstrate the perfusion during the CT scan. In turn, this could guide the perfusion method during the MR scan, avoiding repetitive scans and reducing the duration of MRI.
Although the CT scanning of large specimens has been extensively reported (21,35-37), most of those studies pertain to fresh specimens. Meanwhile, although MRI scanning can provide excellent soft-tissue images, due to the difficulty of acquiring MR images and the long scanning time, there are few related reports in the literature (21); on the contrary, the 3D Slicer platform provides excellent CT and MR registration, and its image editing capabilities can further enhance the value of the MR images.
For CT imaging in our study, we used a SOMATOM Drive Dual-Source CT device (Siemens Healthineers, Erlangen, Germany) with a scanning detector collimation width of 128 mm × 0.6 mm, a frame rotation time of 0.5 s/r, and a scanning layer thickness of 0.75 mm. After the specimen was fixed, the CT image without contrast was first acquired under a tube voltage of 100 kV and 60 mAs. The contrast agent was injected through the aforementioned vessels in stages. After the injection of the contrast agent, enhanced CT scanning was performed.
For MRI acquisition, we used the Discovery 750 3-T MRI device (GE HealthCare, Chicago, IL, USA). The specimen was placed in an eight-channel head coil and scanned using the sagittal time-resolved imaging of contrast kinetics (TRICKS) sequence. The sequence parameters were as follows: scanning field, 260 mm × 260 mm; layer thickness, 1 mm; flip angle, 20°; matrix, 384 × 320; repetition number, 1; repetition time (TR) 3.2 ms with a minimum echo time (TE) value; and K-space filling, center-first filling. Similarly, MR images without contrast injection and MR images after contrast injection were acquired. The final step involved reconstructing the vessel in the 3D Slicer image computing platform by using DICOM files to obtain a 3D image of the specimen’s blood vessels and further editing and optimizing the blood vessels and brain tissue.
In addition, for further cost savings, the contrast agents used in this experiment were clinically expired contrast agents, which can be recycled from the neuroradiology department and used to facilitate the workflow for any anatomical laboratory run with limited resources. The mixed circulating fluid containing the contrast agent used during the examination could be recovered at the end of the examination and a more clarified fluid could be obtained by filtering out the tissue residue. Moreover, these contrast agents could be applied in subsequent experiments since they had a wide concentration range for visualization.
Reconstruction of 3D models
We used a medical digital imaging program, the Picture Archiving and Communication System (PACS), to transmit and collect data and loaded the images onto a standard computer (CPU: Intel Core i7-4960X, Memory: 8 GB, hard drive: 120 GB, GPU: Nvidia Quadro K2200 4 GB; Nvidia, Santa Clara, CA, USA) for postprocessing. The 3D surface modeling of anatomical structures was performed with the 3D Slicer software platform (38). 3D Slicer or simply Slicer is a medical image computing platform in global clinical research and is primarily funded by the United States.
The acquisition of DICOM images was performed by a senior radiologist, and the reconstruction of the 3D model of the brain specimen was carried out by a master’s student in neurosurgery with basic knowledge of the techniques of using the 3D reconstruction software under the guidance of a senior neurosurgeon with extensive anatomical experience. The generation process was roughly divided into three steps. First, a specific sequence was selected, and different structures were extracted using an editor based on different densities/signals. T1 or T2 sequences are commonly utilized for reconstructing brain tissues, whereas CT sequences are employed for reconstructing the skull. After the injection of contrast agents, enhanced sequences are used for vascular reconstruction. Notably, different modalities can be registered to the same position using the “Registration Module” in 3D Slicer software. In this initial step, the “Threshold” and “Flood Filling” functions in the “Segment Editor Module” are typically employed to create a preliminary, relatively coarse model. Second, optimization was performed from the segmented structures to eliminate interfering information and retain the target information. A variety of tools can be used for this purpose, such as the “Paint” tool for adding information, the “Erase” or “Scissors” tools for trimming, and the “Smoothing” tool for smoothing. The selection of these tools is often influenced by personal preference and the specific needs of the segmentation. Third, the model was generated according to the anatomical requirements (Figure 4). Each basic model was completed in approximately 0.5–1 hour from start to finish, whereas more detailed models could require slightly more time.
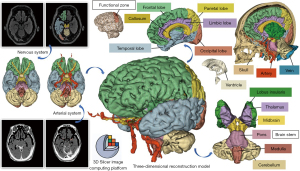
For aged specimens, the leakage of contrast agent is inevitable. Generally, the display of the contrast agent within the 3D Slicer software is high-threshold. The contrast agent within the blood vessels commonly shows a continuous high-threshold display, which usually aligns with our anatomical understanding. Therefore, we can read the slices and trace from the proximal to the distal end of the blood vessels to identify the leakage sites. With the combination of tools in the 3D Slicer software mentioned in the second step of the previous paragraph, we can modify the segmentations to retain the correct blood vessels. In addition, we observed that arterial leakage is less frequent than venous leakage due to the differences in the thickness of the blood vessel walls. Consequently, it is advisable to complete the arterial system scan before injecting the contrast agent into the venous system.
Results
3D reconstruction of isolated specimens was completed through digital cranio-cerebral reconstruction combined with the perfusion scanning of blood vessels. It was observed that part of the intracranial blood vessels, along with other soft tissues, could be accurately reconstructed with high precision. Moreover, the fusion of multimodal images facilitated the 3D Slicer platform in restoring the internal anatomical structure of the specimen.
We found that vascular perfusion was better visualized in the CT modality than in the MRI modality, whereas soft tissues such as brain parenchyma were better visualized in the MRI modality. Additionally, high-quality reconstruction of the 3D model could be accomplished by leveraging the advantages of both modalities. Blood vessels in aged specimens had different degrees of blockage and leakage, which is not a problem encountered in fresh specimens. For instance, the arterial segment passed through the ICA and reached the M1–M3 segment of the middle cerebral artery, the VA could also reach the P1–P3 segment, the anterior cerebral artery occasionally reached the A2 segment, and the ophthalmic artery could be observed in some specimens. The venous segments passed from as far as the sigmoid sinus to reach the superior sagittal sinus through the transverse sinus, whereas leakage from the veins was particularly severe and often resulted in contrast permeation to various locations within the skull after the venous segment had completed perfusion, resulting in poor image quality (Figure 5). All those aspects require appropriate countermeasures at the time of image post-processing.
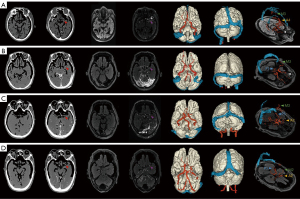
Discussion
Enhanced utilization of aged anatomical specimens
This study established an easy, fast, and residue-free vascular reconstruction model for brain specimens, regardless of their postmortem age. In the preliminary experiments, we demonstrated that the morphology of soft tissues, such as brain tissue, can be largely maintained in aged specimens, making them perpetually available as digital twins. This is because our stepwise guide addresses the issue of blood vessels being typically collapsed due to blood loss in aged specimens: in fact, by reconstructing and filling the blood vessels, the structure of the specimen can be optimally restored. The main steps of the modeling include preprocessing the specimen, injecting a reusable contrast agent to acquire image data, and performing 3D reconstruction based on the image data.
The contrast agents used in the experiments are common, readily available, and simple to configure. These contrast agents, including iopromide and gadodiamide, are commonly used angiographic agents in the clinic and are low-cost and reusable. These contrast agents have a wide concentration range for visualization and therefore have a large margin for error in their configuration. The injection process is simple and fast, and the contrast agents are colorless and transparent and do not contaminate or damage the specimen.
We chose not to use contrast-containing latex for vascular perfusion (39) or advanced contrast media based on nanopolymers of hyaluronic acid (40) based on the following considerations:
- Aged specimens have many impurities in the vessels, such as thrombus and tissue debris, and perfusion with solids may result in a buildup of impurities that do not allow for the distal vessels to be visualized;
- Aged specimens have weak or broken vessel walls and are more gently perfused with fluid;
- Diluted contrast solutions are low-cost and recyclable;
- The contrast solution does not require heating and is easy to configure.
For fresh specimens, the quality of vessel preservation is high and amenable to reconstruction. However, the vascular condition of aged specimens is poor, and vascular damage may occur in each segment, resulting in the extravasation of the contrast agent, which makes reconstruction more difficult. With the powerful editing function of 3D Slicer software, we can edit and optimize the extravasation portion, deriving accurate information even from aged specimens.
Although CT scans of large specimens are more common than MRI scans, MRI provides better-quality images of soft tissue. Despite cold temperatures potentially reducing the contrast of the image (41), MRI still provides more information than a conventional CT scan. Formalin fixation hardens the specimen and is not conducive to high-fidelity surgical simulation (22), but the digitized images of old specimens serve as a significant adjunct to supplemental teaching in medical courses.
Application of the proposed method to fresh specimens
While completing the experiments on aged specimens, we compared the differences between fresh and aged specimens and attempted to apply the vascular reconstruction model described above to fresh specimens. For example, we performed CT and MRI scans of porcine livers (39,42) (Figure 6) and porcine kidneys (43) (Figure 7). The results showed that CT scans outperformed MRI scans in terms of vascular reconstruction after angiographic contrast agent perfusion. In addition, 3D reconstruction with 3D Slicer performed well for large vessels but was more inadequate for microvessels (Figures 6F,7F).
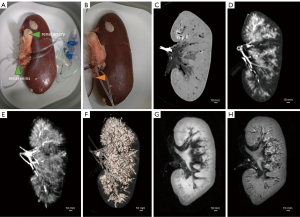
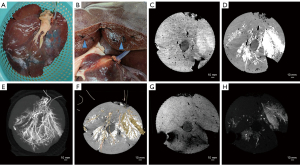
We also attempted to apply this model to porcine head specimens to simulate the perfusion of basal ganglia feeders (such as medial and lateral lenticulostriate, and anterior choroidal arteries), which are known to be extremely small. In our experimental setting, we found that the intracranial blood vessels in pigs were too small, leading to difficulty in delivering the contrast agent into the intracranial blood vessels and obtained an unsatisfactory perfusion (Figure 8). This suggests that our method, despite all the advantages listed above, has certain limitations in terms of the internal diameter of the vessels. Considering the results of previous experiments on aged specimens, we concluded that when the inner diameter of the vessel is less than 1 mm, it may affect the perfusion effect and make the reconstruction of microvessels difficult.
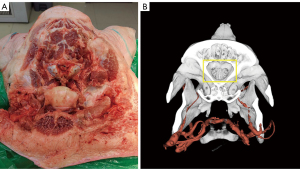
Therefore, special attention needs to be paid to the size of the vessels when applying this vascular reconstruction model. For large vessels, the method performs well; whereas for microvessels, especially if the internal diameter of the vessel is less than 1 mm, the results may be less than optimal. This finding helps us to select specimens more precisely when applying the technique and to optimize the perfusion and reconstruction process for better overall results. Therefore, by applying the technique to fresh specimens, we not only verified the applicability of the reconstruction model but also clarified its advantages and limitations under specific vessel internal diameters, thus providing a valuable reference for further technical improvement and application.
Combined application of instructional anatomical specimens with multimodal images
Anatomical images are of paramount importance to the instruction of anatomy, and the use of digitized human specimens is gradually growing. Meanwhile, the emergence of increasingly sophisticated mathematical models and image-processing and visualization tools has made it possible to present anatomically complex structures in 3D.
In terms of long-term applications, the digitization of specimens allows for the scanning of specimens prior to dissection, which can then be combined with multimodal images to reconstruct a more realistic and comprehensible 3D image. This not only preserves more anatomical information, but also allows for the depiction of structures that might have been damaged or destroyed during dissection (25). Effectively, our study demonstrates that it is possible to introduce a paradigm shift in the use of anatomical specimens, which are not to be considered a “disposable” learning tool anymore and can become a perpetual resource instead. These digital models allow students to perform pre-anatomy previews, intra-anatomy comparisons, and post-anatomy reviews in a manner similar to that of a surgeon’s preoperative reading of CT or MRI images and 3D reconstruction for surgical planning, which is more in line with the clinical mindset of reading and learning (Figure 9A,9B).
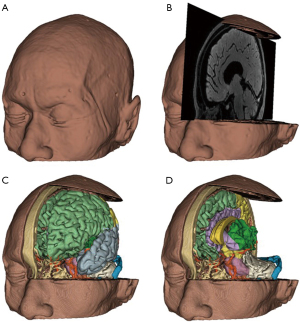
The current use of anatomical specimens primarily revolves around dissection after fixation. However, the loss of intravascular fluid after the specimen is dissected results in vascular collapse, and if CT or MR scans are performed directly, the integrity and accuracy of the internal tissue images of the specimen may be lacking. With the model described in this paper, it is possible to fill the vessels within the specimen and reconstruct them after rendering them visible via a contrast agent that does not require heating, which also helps in the determination of the intracranial tissue structure. This proposed method, compared to the traditional latex perfusion method, has the advantages of simplicity, speed, and the absence of residue.
In the case of aged specimens, the contrast-containing fluid injected into the internal vasculature may leak out into the surrounding tissues due to the nature of the specimen itself or due to an extensive storage time, thus affecting visualization. However, by using the powerful editing capabilities of the 3D Slicer software platform, unnecessary or interfering portions can be trimmed to highlight the desired anatomical structures, thus enhancing the specimen’s value in education or research. Moreover, when provided with the digital information of the specimen, students will be able to conduct their own editing and optimization using the 3D Slicer software, offering a new dimension in anatomy education (8).
The model is not only suitable for brain specimens, but can also be applied to other biological or nonbiological specimens, such as cardiac coronary arteries. Through injection of a contrast-containing solution into the target lumen or duct and completion of a CT or MRI scan, the contrast agent causes the solution to contrast sharply with the surrounding tissues. Subsequently, a multimodal 3D image can be obtained through the reconstruction of the scanned image, which can satisfy a variety of medical needs and has a wide range of applications.
In summary, advances in imaging technology have enabled us to visualize anatomical structures in greater detail and multiple dimensions. With the help of computer technology and postprocessing software, traditional specimens can be digitally reconstructed and preserved with greater ease. The technique of combining 3D reconstruction with multimodal images makes the learning of anatomical structures more realistic, intuitive, and efficient.
Digital virtual neurosurgery is now available as a method of training residents and as a preoperative tool for practicing neurosurgeons (14,27,31). In the past, neurosurgeons typically reconstructed 3D views of anatomical structures in their minds based on information from 2D CT and MRI scan slices (17) (Figure 9A,9B); in contrast, the modern-day visual 3D image approach allows neurosurgeons to perceive anatomical landmarks and lesion descriptions in advance through a virtual surgical window with a 3D perspective, rendering mental visualization obsolete (4,28) (Figure 9C,9D). It is believed that the greater integration of digital virtual technology and multimodal images will significantly improve the anatomical learning process and the safety of neurosurgery.
Conclusions
This paper outlines a simple digital reconstruction model for brain specimens. By slowly and continuously injecting fluid into the specimen’s blood vessels for reperfusion and then injecting a contrast agent for CT or MRI scanning, we could obtain DICOM images for vascular reconstruction. After combining the CT and MR images into multimodal image data, we employed the open-source 3D Slicer software platform to reconstruct and optimize the images in three dimensions, finally generating a digital 3D reconstruction model of the cranial brain specimen. The model can provide vivid, intuitive 3D images for observers’ reference. It can be applied to the digital preservation of specimen information, medical anatomy instruction, and surgical training and thus holds considerable application value.
Acknowledgments
None.
Footnote
Funding: This study was supported by
Conflicts of Interest: All authors have completed the ICMJE uniform disclosure form (available at https://qims.amegroups.com/article/view/10.21037/qims-24-2301/coif). M.G. serves as an unpaid editorial board member of Quantitative Imaging in Medicine and Surgery. M.G. reports his leadership roles in Ethico-Legal Committee EANS and Oxford Global Neurosurgery Initiative. The other authors have no conflicts of interest to declare.
Ethical Statement: The authors are accountable for all aspects of the work in ensuring that questions related to the accuracy or integrity of any part of the work are appropriately investigated and resolved. The study was conducted in accordance with the Declaration of Helsinki (as revised in 2013). Additionally, the study was conducted with the consent of the donors and their families, in accordance with articles 1, 3, and 5 of the Administrative Measures of Guangzhou Municipality on Voluntary Body Donation. The requirement for ethical approval was waived by the Ethics Committee of the Third Affiliated Hospital of Sun Yat-sen University as the individuals had willingly donated their bodies for scientific purposes.
Open Access Statement: This is an Open Access article distributed in accordance with the Creative Commons Attribution-NonCommercial-NoDerivs 4.0 International License (CC BY-NC-ND 4.0), which permits the non-commercial replication and distribution of the article with the strict proviso that no changes or edits are made and the original work is properly cited (including links to both the formal publication through the relevant DOI and the license). See: https://creativecommons.org/licenses/by-nc-nd/4.0/.
References
- Jacquesson T, Simon E, Dauleac C, Margueron L, Robinson P, Mertens P. Stereoscopic three-dimensional visualization: interest for neuroanatomy teaching in medical school. Surg Radiol Anat 2020;42:719-27.
- Ghosh SK. Cadaveric dissection as an educational tool for anatomical sciences in the 21st century. Anat Sci Educ 2017;10:286-99.
- Turkoglu E, Seckin H, Gurer B, Ahmed A, Uluc K, Pulfer K, Arat A, Niemann D, Baskaya MK. The cadaveric perfusion and angiography as a teaching tool: imaging the intracranial vasculature in cadavers. J Neurol Surg B Skull Base 2014;75:435-44.
- Oliveira ASB, Leonel LCPC, Bauman MMJ, De Bonis A, LaHood ER, Graepel S, Link MJ, Pinheiro-Neto CD, Lachman N, Morris JM, Peris-Celda M. Photogrammetry scans for neuroanatomy education - a new multi-camera system: technical note. Neuroinformatics 2024;22:317-27.
- Tajika Y, Ishii N, Morimura Y, Fukuda K, Shikada M, Murakami T, Ichinose S, Yoshimoto Y, Iwasaki H. Correlative microscopy and block-face imaging (CoMBI): a 3D imaging method with wide applicability in the field of biological science. Anat Sci Int 2023;98:353-9.
- Gurses ME, Hanalioglu S, Mignucci-Jiménez G, Gökalp E, Gonzalez-Romo NI, Gungor A, Cohen-Gadol AA, Türe U, Lawton MT, Preul MC. Three-Dimensional Modeling and Extended Reality Simulations of the Cross-Sectional Anatomy of the Cerebrum, Cerebellum, and Brainstem. Oper Neurosurg (Hagerstown) 2023;25:3-10.
- Oliveira ASB, Fernandes JVA, Figueiredo VLFA, Leonel LCPC, Bauman MMJ, Link MJ, Peris-Celda M. 3D Models as a Source for Neuroanatomy Education: A Stepwise White Matter Dissection Using 3D Images and Photogrammetry Scans. Brain Topogr 2024;37:947-60.
- Spitzer V, Ackerman MJ, Scherzinger AL, Whitlock D. The visible human male: a technical report. J Am Med Inform Assoc 1996;3:118-30.
- Lai M, Skyrman S, Kor F, Homan R, El-Hajj VG, Babic D, Edström E, Elmi-Terander A, Hendriks BHW, de With PHN. Development of a CT-Compatible, Anthropomorphic Skull and Brain Phantom for Neurosurgical Planning, Training, and Simulation. Bioengineering (Basel) 2022;9:537.
- Balogh AA, Preul MC, László K, Schornak M, Hickman M, Deshmukh P, Spetzler RF. Multilayer image grid reconstruction technology: four-dimensional interactive image reconstruction of microsurgical neuroanatomic dissections. Neurosurgery 2006;58:ONS157-65; discussion ONS157-65.
- de Notaris M, Prats-Galino A, Cavallo LM, Esposito F, Iaconetta G, Gonzalez JB, Montagnani S, Ferrer E, Cappabianca P. Preliminary experience with a new three-dimensional computer-based model for the study and the analysis of skull base approaches. Childs Nerv Syst 2010;26:621-6.
- Pujol S, Baldwin M, Nassiri J, Kikinis R, Shaffer K. Using 3D Modeling Techniques to Enhance Teaching of Difficult Anatomical Concepts. Acad Radiol 2016;23:507-16.
- Martínez Santos JL, Aljuboori Z, Richardson AM, Hanalioglu S, Peker HO, Aydin I, Al-Sharshahi ZF, Tunc O, Karatas D, Avci E, Baskaya MK. Microsurgical anatomy and approaches to thalamic gliomas. Part 1: A cartography guide for navigating to the thalamus. Integrating 3D model rendering with anatomical dissections. J Neurosurg 2024;141:1457-71.
- Aridan N, Bernstein-Eliav M, Gamzo D, Schmeidler M, Tik N, Tavor I. Neuroanatomy in virtual reality: Development and pedagogical evaluation of photogrammetry-based 3D brain models. Anat Sci Educ 2024;17:239-48.
- Patra A, Pushpa NB, Ravi KS. Visualization in Anatomy Education. Adv Exp Med Biol 2023;1406:171-86.
- Zhao JC, Chen C, Rosenblatt SS, Meyer JR, Edelman RR, Batjer HH, Ciric IS. Imaging the cerebrovascular tree in the cadaveric head for planning surgical strategy. Neurosurgery 2002;51:1222-7; discussion 1227-8.
- Rasmussen AS, Lauridsen H, Laustsen C, Jensen BG, Pedersen SF, Uhrenholt L, Boel LW, Uldbjerg N, Wang T, Pedersen M. High-resolution ex vivo magnetic resonance angiography: a feasibility study on biological and medical tissues. BMC Physiol 2010;10:3.
- Krogager ME, Dahl RH, Poulsgaard L, Fugleholm K, Sehested T, Mikkelsen R, Tranum-Jensen J, Mathiesen TI, Benndorf G. Combined cone-beam CT imaging and microsurgical dissection of cadaver specimens to study cerebral venous anatomy: a technical note. Surg Radiol Anat 2023;45:1177-84.
- Pan SQ, Chan LK, Yan Y, Yang X. Survey of Gross Anatomy Education in China: The Past and the Present. Anat Sci Educ 2020;13:390-400.
- Bruguier C, Egger C, Vallée JP, Grimm J, Boulanger X, Jackowski C, Mangin P, Grabherr S. Postmortem magnetic resonance imaging of the heart ex situ: development of technical protocols. Int J Legal Med 2015;129:559-67.
- Grabherr S, Grimm J, Baumann P, Mangin P. Application of contrast media in post-mortem imaging (CT and MRI). Radiol Med 2015;120:824-34.
- Aboud E, Al-Mefty O, Yaşargil MG. New laboratory model for neurosurgical training that simulates live surgery. J Neurosurg 2002;97:1367-72.
- Grabherr S, Grimm J, Dominguez A, Vanhaebost J, Mangin P. Advances in post-mortem CT-angiography. Br J Radiol 2014;87:20130488.
- Rosset A, Spadola L, Ratib O. OsiriX: an open-source software for navigating in multidimensional DICOM images. J Digit Imaging 2004;17:205-16.
- Uhl JF, Sufianov A, Ruiz C, Iakimov Y, Mogorron HJ, Encarnacion Ramirez M, Prat G, Lorea B, Baldoncini M, Goncharov E, Ramirez I, Céspedes JRC, Nurmukhametov R, Montemurro N. The Use of 3D Printed Models for Surgical Simulation of Cranioplasty in Craniosynostosis as Training and Education. Brain Sci 2023;13:894.
- Aydin SO, Barut O, Yilmaz MO, Sahin B, Akyoldas G, Akgun MY, Baran O, Tanriover N. Use of 3-Dimensional Modeling and Augmented/Virtual Reality Applications in Microsurgical Neuroanatomy Training. Oper Neurosurg (Hagerstown) 2023;24:318-23.
- Erol G, Güngör A, Sevgi UT, Gülsuna B, Doğruel Y, Emmez H, Türe U. Creation of a microsurgical neuroanatomy laboratory and virtual operating room: a preliminary study. Neurosurg Focus 2024;56:E6.
- de Notaris M, Topczewski T, de Angelis M, Enseñat J, Alobid I, Gondolbleu AM, Soria G, Gonzalez JB, Ferrer E, Prats-Galino A. Anatomic skull base education using advanced neuroimaging techniques. World Neurosurg 2013;79:S16.e9-13.
- Bulla A, Casoli C, Farace F, Mazzarello V, De Luca L, Rubino C, Montella A. A new contrast agent for radiological and dissection studies of the arterial network of anatomic specimens. Surg Radiol Anat 2014;36:79-83.
- Kingston MJ, Perriman DM, Neeman T, Smith PN, Webb AL. Contrast agent comparison for three-dimensional micro-CT angiography: A cadaveric study. Contrast Media Mol Imaging 2016;11:319-24.
- Piazza A, Spiriev T, Corvino S, Corrivetti F, Laleva L, Iaconetta G, de Notaris M. The Course of the Trochlear Nerve Presented via a 3-Dimensional Photorealistic Anatomic Model. World Neurosurg 2024;186:e156-60.
- Sanan A, Abdel Aziz KM, Janjua RM, van Loveren HR, Keller JT. Colored silicone injection for use in neurosurgical dissections: anatomic technical note. Neurosurgery 1999;45:1267-71; discussion 1271-4.
- Grabherr S, Djonov V, Yen K, Thali MJ, Dirnhofer R. Postmortem angiography: review of former and current methods. AJR Am J Roentgenol 2007;188:832-8.
- Blery P, Pilet P, Bossche AV, Thery A, Guicheux J, Amouriq Y, Espitalier F, Mathieu N, Weiss P. Vascular imaging with contrast agent in hard and soft tissues using microcomputed-tomography. J Microsc 2016;262:40-9.
- Ruder TD, Thali MJ, Hatch GM. Essentials of forensic post-mortem MR imaging in adults. Br J Radiol 2014;87:20130567.
- Pommert A, Höhne KH, Burmester E, Gehrmann S, Leuwer R, Petersik A, Pflesser B, Tiede U. Computer-based anatomy a prerequisite for computer-assisted radiology and surgery. Acad Radiol 2006;13:104-12.
- Nikolaou K, Becker CR, Muders M, Babaryka G, Scheidler J, Flohr T, Loehrs U, Reiser MF, Fayad ZA. Multidetector-row computed tomography and magnetic resonance imaging of atherosclerotic lesions in human ex vivo coronary arteries. Atherosclerosis 2004;174:243-52.
- Bruns N. 3D Slicer: Universal 3D visualization software. Unfallchirurg 2019;122:662-3.
- Renard Y, Hossu G, Chen B, Krebs M, Labrousse M, Perez M. A guide for effective anatomical vascularization studies: useful ex vivo methods for both CT and MRI imaging before dissection. J Anat 2018;232:15-25.
- Ganau M, Syrmos NC, D'Arco F, Ganau L, Chibbaro S, Prisco L, Ligarotti GKI, Ambu R, Soddu A. Enhancing contrast agents and radiotracers performance through hyaluronic acid-coating in neuroradiology and nuclear medicine. Hell J Nucl Med 2017;20:166-8.
- Matsumoto M, Kodama N, Endo Y, Sakuma J, Suzuki K, Sasaki T, Murakami K, Suzuki K, Katakura T, Shishido F. Dynamic 3D-CT angiography. AJNR Am J Neuroradiol 2007;28:299-304.
- Xiao J, Cui Z, Fu M, Kong X, Tang L, Wang Z, You F, Du Q, Li J. An ex vivo liver training model continuously perfused to simulate bleeding for suture skills involved in laparoscopic liver resection: development and validity. Surg Endosc 2016;30:4553-61.
- Jorgensen SM, Demirkaya O, Ritman EL. Three-dimensional imaging of vasculature and parenchyma in intact rodent organs with X-ray micro-CT. Am J Physiol 1998;275:H1103-14.