Minimally invasive interventional guided imaging therapies of musculoskeletal tumors
Introduction
Soft tissue and bone tumors form the group of musculoskeletal (MSK) tumors, but have been managed as distinct entities. In addition, there are several congenital syndromes that are linked to MSK tumors, such as Beckwith-Wiedemann syndrome (characterized by myxomas, fibromas, and hamartomas), Cowden syndrome (associated with lipomas and hemangiomas), Maffucci syndrome (involving chondroid tumors and hemangiomas), and McCune-Albright syndrome (which is associated with fibrous dysplasia and osteosarcomas). Other syndromes that are related to MSK tumors include neurofibromatosis type I and II disorders, as well as Ollier disease (which is characterized by chondroid tumors), among others. The incidence of benign soft tissue tumors within the first group (soft-tissue tumors), is not well established but is likely to be far higher than that of malignant tumors, with a ratio of approximately 100:1. The categorization of MSK tumors mostly depends on their source (adipocytes, muscle cells, cartilage, etc.) and their propensity for different behaviors (benign, intermediate, or malignant). The occurrence of soft-tissue metastases is few, but their identification has significantly risen as a result of advanced imaging methods such as magnetic resonance imaging (MRI) and positron emission tomography/computed tomography (PET/CT). Bone tumors are the second most widely acknowledged kind of MSK tumors. Metastatic bone tumors are more prevalent than primary bone cancers, such as osteosarcoma, Ewing’s sarcoma, or chondrosarcoma; in fact, the MSK system is a frequently affected location for metastatic disease (1). Autopsy findings indicate that osseous metastases are present in up to 85% of patients diagnosed with breast, prostate, kidney, and lung malignancies (2). The occurrence of metastases, particularly bone metastasis, in individuals with MSK tumors can result in the emergence of significant issues such as discomfort, impairment of vital anatomical structures, or pathological fractures (3). Given these issues, a diverse team of experts is typically engaged in intricate treatment decision-making concerning the necessity of surgical intervention, radiation therapy, chemotherapy, or a combination of these modalities. Nevertheless, percutaneous image-guided ablation is a viable alternative method for treating MSK tumors. Over the past decade, significant progress has been made in this approach, leading to its increased integration into standard clinical practice (4). May be recommended in the following clinical situations: when there is ongoing pain or evidence of tumor growth despite receiving the maximum dose of radiation therapy, when radiation therapy is not possible due to specific reasons such as the need for ongoing systemic therapy, when the patient does not wish to undergo radiation therapy, or when systemic treatments and pain medications have not been effective in treating the condition adequately. Various image-guided methods have been employed to observe the ablation zone and nearby structures, such as fluoroscopy, ultrasonography (US), computed tomography (CT), and MRI. However, CT is the favored choice due to its widespread availability and ability to visualize the tumor and its environs. MRI provides exceptional imaging of MSK tumors, yet there are few options for ablation and bone-access devices that are compatible with this imaging modality. The use of US may have potential benefits in the diagnosis and evaluation of superficial MSK tumors (5). MSK tumors can be treated with ablation procedures, either on their own or in conjunction with other percutaneous techniques. Chemical ablation includes the use of injected ethanol into the tumor. Thermal ablation is a medical procedure that involves using different methods to deposit energy, such as laser, radiofrequency (RF), microwave, cryoablation (CA) and magnetic resonance (MR)-guided high-intensity focused ultrasound (HIFU). The ablation operation can be conducted in tandem with cementation, with or without additional metallic stabilizing devices, depending on the location of the lesion. Improved local tumor control can be attained with the utilization of ablation in conjunction with either bland embolization or trans arterial chemoembolization (6). This article provides an overview of the fundamental elements involved in the image-guided ablation of MSK tumors.
Percutaneous chemical alcohol ablation (PAA)
Percutaneous ethanol injection, also known as alcohol ablation, is a straightforward and cost-effective technique for percutaneous tumor ablation (7,8). The procedure initially involved the percutaneous administration of a sclerosing chemical to treat kidney cysts. The administration of alcohol causes tumor necrosis by means of cellular dehydration and indirectly through arterial thrombosis and tissue ischemia (7,9). Alcohol can be utilized for neurolysis, a procedure that has shown effective in treating neuromas and nerve tumors directly; it induces Wallerian degeneration, protein denaturation, and coagulative necrosis of neural tissue (10,11).
Alcohol injection has demonstrated promise in providing relief for symptomatic bone tumors, including metastases and vertebral hemangiomas. This treatment has been found to reduce pain within 24–48 hours and can provide relief for 2 to 7 months. The success rate of alcohol injection ranges from 70 to 90% (7,12,13). Nevertheless, the predictability of the extent of necrosis caused is low due to the challenging task of anticipating the dispersion of ethanol, such as in vertebral hemangiomas (13,14). A significant drawback of bone injection is its high level of discomfort, often necessitating the use of neuroleptanalgesia. Combining PAA with radiofrequency ablation (RFA) (15-17) can enhance the success rate, and this approach has been applied to treat visceral and soft tissue tumors that are larger than 4 cm. In sum, the primary benefit of alcohol ablation is its cost-effectiveness (18).
Technique
The PAA approach was initially documented by Heiss et al. (19) in the context of vertebral hemangiomas. The technique is typically conducted with the patient under neuroleptanalgesia. An unenhanced CT scan is conducted to evaluate the most suitable location for puncture. Administering iodinated contrast material has been utilized to clearly define the extent of ethanol diffusion in order to reduce the risk of vascular intravasation and possibly unintended damage to nearby vital structures (18). Strict adherence to aseptic conditions is necessary. Following the application of a local anesthetic, a needle with a diameter of 18- to 22-gauge is inserted into the tumor. First, a diluted solution of contrast medium (25%) mixed with lidocaine (75%) is injected into the lesion. Lidocaine is directly injected into the tumor to alleviate the pain caused by the alcohol injection. CT imaging reveals the pattern of contrast agent distribution within the tumor and can forecast the spread of ethanol within the abnormal tissue (3). Ultimately, a volume of 3–10 mL (varying according to the size of the tumor) of 96% ethanol is administered directly into the tumor. An absolute alcohol solution with a concentration of 95–100% and a volume ranging from 3–30 mL is immediately injected into the specific tissue (18). For sizable tumors, it is necessary to provide ethanol into the specific areas that have been pinpointed as the source of pain, typically located at the outer edges. Adjusting the position of the needle can facilitate access to areas with limited dispersion. If the contrast spreads beyond the boundaries of the tumor, especially when it reaches nearby neurological regions, the operation is stopped. To counteract this, an isotonic solution is administered to dilute the alcohol that was previously injected. Vertebral hemangiomas carry a potential danger of retrograde filling of the segmental arteries, which could result in reflux into an artery that supplies blood to the spinal cord. Caution should be exercised when administering the injection if the artery of Adamkiewicz arises at the same level as the hemangioma (20). Other potential application of PAA is sclerosing the Morton’s neuroma of the foot. In this case, Pabinger et al. suggest using electroneurographic confirmation to evaluate its efficacy (21).
Indications
A classical indication of PAA emerged from the treatment of osteoid osteoma. The initial description of this phenomenon was provided in 1989 by Doyle et al. (22), with the primary objective being the complete eradication of the nidus (9,23,24). PAA is generally performed in conjunction with the drilling of the nidus. Prior to treatment, it is advisable to get a biopsy in order to verify the diagnosis and rule out the presence of other etiologies such as chondroblastoma, Brodie’s abscess or eosinophilic granuloma. Given that the majority of patients with osteoid osteoma are prescribed acetylsalicylic acid, it is advised to cease the medication one week before to the treatment (24). The success rates exceed 90% with minimal recurrence and complication rates (17,22-24). Another original indication was the treatment of aggressive vertebral hemangiomas, which can be coupled with surgery and/or cementoplasty to decrease the risk of bleeding during surgery (25). PAA is recommended too for patients with painful and severe osteolytic bone metastases who have not responded to traditional anticancer therapy and have poor medical care (1,26), or in situations where prompt pain alleviation is necessary, considering the typical time lag of 2–4 weeks following radiation therapy or chemotherapy. Over the past few decades, the predictability of ablation volumes has significantly improved with the use of thermal ablation techniques. As a result, PAA has been considered less important and has been given a lower priority. Morton’s neuroma is another non-threatening condition that can be treated. However, if conservative treatment is unsuccessful, corticosteroid injection yields superior outcomes. In this scenario, the procedure can be carried out using local anesthesia (21,27-30). Sanders et al. (31) employed PAA as a treatment for a superficial intramuscular glomus tumor. According to the authors, the tumor’s soft, vascular, and encapsulated characteristics facilitated controlled spreading, offering a non-invasive option to surgical intervention. Finally, ethanol can be utilized for conducting regional nerve block in individuals experiencing intense and unmanageable pain due to tumors. The study conducted by Gangi et al. (26) utilized CT to provide guidance for various types of neurolytic blocks, including those targeting the spinal nerve, sympathetic chain, pterygopalatine ganglion, brachial plexus, and celiac plexus.
Contraindications
The most important contraindication is a risk of ethanol diffusion into vital structures (26). Uncorrectable coagulopathy and allergy to local anesthesia or ethanol are also contraindications.
Complications
The global risk profile of PAA in visceral tumors has been extensively researched. A prior investigation, which included 746 individuals diagnosed with hepatocellular carcinoma, revealed that 1.7% of the medical interventions resulted in significant problems (32). Potential consequences of the operation include neurolysis, which is the destruction of nerve tissue, and extensive tumor necrosis, which is the death of tumor cells, accompanied by fever and hyperuricemia, which is an elevated level of uric acid in the blood. Within the initial 72-hour period, Gangi (26) documented a mild increase in body temperature in 11 individuals. Following the injection of alcohol into the affected area, a patient with spinal metastasis experienced paraparesis, along with severe neuralgia at C-5, C-6, and C-7. The metastasis had spread into the brachial plexus. Excessive ethanol consumption can cause aseptic necrosis and pathological fracture in spinal lesions. Several studies suggest a volume restriction of 15 mL, since it effectively accomplishes the intended therapeutic outcome without causing pathological fractures (21).
Less often documented problems include infection and hemorrhaging (13). Due to its properties as a non-ionic contrast medium, it provides an ideal environment for the growth of bacteria. Therefore, certain authors suggest the use of antibiotic prophylaxis (33). The practitioner should consider the possibility of systemic hypotension, elevated pulmonary vascular resistance, and myocardial toxicity. They should also be mindful of the probable requirement to address bouts of hypotension after PAA. Nevertheless, these problems are infrequently observed in the context of alcohol embolization administered intraarterially. For summarize, although PAA is associated with certain complications, it has been proven to be a significantly safer and more efficient treatment compared to simple surgical excision (7,9,12,32). However, it is important to note that one of the main drawbacks of PAA is the unpredictable and inconsistent diffusion of alcohol through tumor and surrounding tissues, which can result in an uncertain geometry and extent of the ablation zone, as well as the potential risk of skin necrosis (18).
RFA
Background and physical principles
RFA is considered the predominant technique employed for thermal ablation of bone and soft tissue malignancies (34,35). RFA refers to the initiation of coagulation through the use of electromagnetic energy from any source with a frequency below 30 MHz (36). The integration of an electrosurgical equipment, known as “Bovie’s Knife”, in 1926 marked the initial introduction of RF into modern medicine. Harvey Cushing initially employed this device in neurosurgical procedures to provide both tissue cauterization and cutting by altering the RF current (37). In 1990, McGahan et al. (38) and Rossi et al. (39) reported on the utilization of RF energy to eliminate liver tumors. The ablation approach relies on the utilization of an alternating electric current applied to the target tissue using an interstitial electrode. The present situation involves the rapid oscillation of ions in the tissue, resulting in the generation of areas with high current density adjacent to the electrode, which in turn leads to the production of frictional heat (37). To comprehend the constraints of RFA, it is imperative to take into account several facets pertaining to its method of action. Firstly, the effectiveness of the process relies heavily on the efficient conduction of electricity and heat through the tissue. However, this conduction is hindered and restricted over time due to the existence of water vapor, desiccation, and charring, which are byproducts of the process (40). The manifestation of these constraining elements is contingent upon the timing of energy application: the more expeditious the timing, the more promptly these factors emerge. Hence, a gradual and incremental transfer of energy is more efficient than a sudden increase in temperature. Furthermore, the rate of conductive heat transfer is often sluggish in the majority of tissues, which therefore restricts the capacity to mitigate other simultaneous processes like perfusion and ventilation. The phenomenon known as the “heat sink” or “cold sink” effect involves the dispersion of thermal energy caused by the natural flow of neighboring vessels with a diameter greater than 2–3 mm (41). The distance from the electrode is a crucial element. The ablating heat originates from the friction generated by the oscillating molecules caused by the electric current. As the distance between the electrode and the molecules grows, the molecular vibration diminishes, leading to an exponential decrease in energy and a consequent decrease in temperature. Goldberg et al. (42) presented a theoretical equation that succinctly explains the primary forces at play in RFA and how they interact; induced coagulation necrosis can be calculated by subtracting heat loss from the product of energy deposited and local tissue interactions. Regarding MSK lesions, the nearby bone, particularly when it thickens due to inflammation, serves as a natural obstacle that hinders the dissipation of energy (43). Overall, the aforementioned characteristics render RFA an optimal choice for the treatment of small soft-tissue lesions that are encircled by bone, such as osteoid osteoma, chondroblastoma, or osteoblastoma (Table 1).
Table 1
Advantages of RFA | Disadvantages of RFA |
---|---|
• Lengthy track record of accumulated data | • Concern regarding needle tract seeding |
• Extensive RFA awareness among oncologists | • Grounding pad-related skin burns |
• Large experience with RF in other | • Heat sink effect may decrease RFA effectivity near great or medium vessels |
• Medical fields (cardiac ablations, electrocautery...) | • Size limitation: RFA of larger lesions can be difficult (from 5–7 cm) |
• Wide availability | • Speed: ablations need to be relatively slow in order to avoid tissue charring or vaporization |
• Relatively cheaper cost compared with newer ablation modalities | • Intraprocedural monitoring with ultrasound may be limited by gas formation, which worsens visualization |
• Small electrode size (14–21 G) | • Operator-dependent technique |
• RFA data in multiple organs | • Dependent on additional imaging guidance |
• Acceptable safety profile | • Inability to visualize the treatment volume real-time (11,18,44) |
• Hemostatic effect, which can be helpful in periprocedural bleeding | |
• MRI-compatible devices available | |
• Typically, single procedure |
RFA, radiofrequency ablation; RF, radiofrequency; MRI, magnetic resonance imaging.
Technical aspects: instrumentation
The RFA device consists of the following components (36,37):
An electric generator produces the electrical current that is utilized by the electrode. Currently, the majority of accessible devices have the ability to provide outputs ranging from 200 to 250 W. These outputs consist of high-frequency alternating current in the range of 375–500 kHz.
Grounding or dispersion pads are typically positioned on the patient’s thighs to avoid electrical continuity.
An electrode is a metallic probe with a gauge size of 14–21 G. It is coated with an insulating substance, except for the tip, which has an active length of 0.7–3 cm. It serves as the cathode in the electrical circuit, which helps confine tissue damage. Various electrode modifications have been devised to accommodate diverse lesion forms and sizes:
- Monopolar electrodes refer to the traditional rod-shaped electrodes that have a heat distribution around their tip. This heat distribution follows a cylindrical contour with rounded edges.
- Multi-tined expandable arrays consist of several curving uninsulated prongs that are arranged in the shape of an umbrella, extending from the needle tip central cannula.
- Internally cooled electrodes: a chilled saline solution is circulated through the needle’s chamber shaft, which leads to a decrease in charring and impedance, as well as an increase in the volume of tumor ablation (45).
- Perfusion electrodes: Saline or hypertonic saline is introduced or administered into the targeted ablation tissue, thereby increasing the size of the tumor ablation by altering the electrical conductivity of the tissue (achieving diameters of up to 7 cm) (46). Currently, it is advised against using normal saline for the purpose of conducting RFA (47).
- Bipolar electrodes: multiple bipolar electrodes are inserted into the tumor and RF energy is delivered between them. By activating all the electrodes within the tumor, we can ensure low energy loss, resulting in larger and more efficient ablation volumes. This approach also helps to prevent the danger of skin pad burns (48).
Procedure
RFA involves the use of a high-frequency alternating continuous current to apply heat to specific tissue using an active electrode tip. This is typically done under the guidance of CT imaging, and it results in the generation of heat and coagulative necrosis in the targeted tissue (11,18,35,44). The goal of RFA is to achieve temperatures ranging from 50–100 ℃ for a duration of 4–6 minutes in the specific area of interest, without causing the formation of vapor or charring. This process aims to create an ablation zone that encompasses the entire tumor and includes a safety margin of surrounding healthy tissue measuring 0.5–1 cm (35). Temperature sensors are typically located at or near the probe tip to monitor tissue temperatures during the process and ensure sufficient heating (35). For an ideal RFA, the recommended tumor size is 3 cm or smaller (37). The procedure is painful, thus, patients typically necessitate general anesthesia or moderate to profound sedation with sufficient analgesic support. The initial step involves imaging of the entire lesion, which is essential for determining the most suitable approach path that avoids delicate anatomical elements like blood arteries or nerves. A needle is introduced into the muscle at the chosen region to serve as a guide for the vertebroplasty needle, which is then positioned in the desired spot. Typically, a biopsy trephine is inserted prior to the ablation procedure to collect tissue from the lesion for pathological examination. Subsequently, the electrode is positioned in the desired area, ensuring that the guiding cannula is removed to prevent any interaction with the active electrode tip. Such interaction could result in the heating and harm of nearby tissues. The generator’s power output is subsequently raised to 90 ℃, and a dry ablation process is carried out for a duration of 6 minutes. Based on the magnitude of the lesion, the electrode can be relocated to a different location, and the treatment can be repeated as necessary. A standard RFA treatment yields an output power of less than 10 W, accompanied with an impedance below 150 Ω. Upon finishing the therapy, the RF electrode is removed, and a dressing is put to the skin (36).
Indications of RFA in MSK tumors
Osteoid osteoma
Osteoid osteoma is a noncancerous bone tumor that is typically found in the lower limbs of adolescents and young adults. Although it is not malignant, it can cause significant pain. It is a frequently occurring condition, making up around 10% of benign bone tumors. Osteoid osteomas often exhibit minimal or negligible development capacity and rarely exceed a diameter of 1.5 cm (18,49). While several untreated osteoid osteomas may exhibit a tendency towards spontaneous regression (50), there are cases where it can lead to significant pain and discomfort, necessitating a therapeutic intervention. Traditionally, the only choices available for treating this condition were surgery (resection and curettage) or conservative therapy using nonsteroidal anti-inflammatory drugs. Nevertheless, in many instances, the identification of the tumor was challenging or necessitated extensive bone removal, indicating a significant likelihood of recurrence and fracture (51), therefore necessitating a minimally invasive treatment method. The technique of RFA for the treatment of osteoid osteoma was initially described by Rosenthal et al. in 1992 (52). Subsequently, numerous investigations have been conducted, yielding quite favorable results. To demonstrate ablation, these investigations necessitated a preliminary diagnosis of osteoid osteoma, which was determined based on the patient’s medical history, clinical examination, and imaging results (Figure 1A,1B). The growing evidence from these investigations demonstrates that RFA possesses the ability to overcome challenges in reaching the affected area and achieve more precise and reliable elimination of the disease, with success rates ranging from 79% to 100% (53-59). Moreover, the thermal insulation property of cortical bone has been verified, demonstrating RFA can be safely conducted in close proximity to the joint surface without causing harm to the cartilage (60). In this point, Tomasian et al. (18) reinforce that intra-articular and spinal osteoid osteomas should be approached with caution due to their close proximity to articular cartilage, subchondral bone plate, spinal cord, and spinal nerve roots. Due to these factors, minimally invasive percutaneous RFA has been established as the most effective treatment for osteoid osteoma (Figure 2). According to the technical recommendation, ablation should be carried out at a 90° angle for a duration of 5–6 minutes. A successful ablation is determined by ensuring that the electrode is positioned in such a way that no part of the lesion is more than 5 mm away from the exposed tip, and that the target temperature is achieved and maintained for the specified time period (18,56,61). Although the recurrence rates with RFA are minimal, there is limited understanding of the underlying causes of recurrence. Several studies (62,63) have revealed evidence indicating that lesions located outside the shaft of a bone are more likely to recur statistically. This is likely because these lesions have less impedance during the RFA operation. In 2018, Shields et al. (59) conducted a retrospective analysis that shown a strong correlation between the maximum diameter of the lucency and sclerotic center of the tumor, and the probability of recurrence.
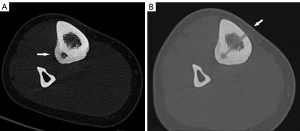
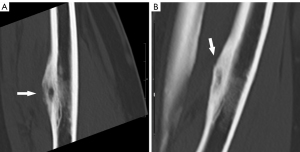
Chondroblastomas
Chondroblastomas are infrequent, noncancerous bone tumors that were initially documented by Jaffe and Lichtenstein in 1942 (64). These tumors constitute around 1% of all non-cancerous bone abnormalities, with 90% of them occurring in individuals within the first three decades of life. The majority of chondroblastomas primarily impact the epiphyses of long bones, causing inflammatory alterations in the neighboring tissues which lead to pain and restricted range of motion (65). Chondroblastomas do not naturally decrease in size without intervention, and as a result, surgical extraction with bone grafting or cementation has traditionally been regarded as the established method of treatment. Nevertheless, surgery is a complex procedure that entails the potential for harm to the articular cartilage or growth plate. While it is generally effective in treating the condition, there is a recurrence incidence of 8% to 35% (66). Recently, RFA has emerged as a viable choice for minimally invasive management of chondroblastoma, offering remarkable pain alleviation rates ranging from 88% to 100%. Additionally, the recurrence rates of 6–12% associated with RFA are much lower than those reported with surgical intervention (67,68). However, the use of RFA in treating chondroblastoma is still not well understood, and the decision on how to approach therapy should be made on an individual basis, taking into account factors such as the location and size of the lesion, as well as the potential negative effects of surgery for each patient (68). The technical specifications for the ablation operations closely resemble those outlined in the RFA of osteoid osteomas. The objective is to eliminate the lesions by subjecting them to a temperature of 90° for a duration of 6 minutes (Figure 3A-3F). Due to their increased size compared to osteoid osteomas, chondroblastomas may require additional ablation procedures to ensure total eradication. Subsequently, it is advisable to undergo clinical and imaging follow-up after the surgery. Despite the little data available due to the rarity of this tumor, it is recommended to have a longer follow-up period as the risk of recurrence is higher in the first 2 years (68-70).
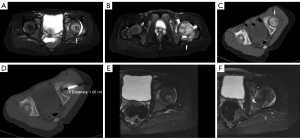
Other benign bone tumors
The information regarding the use of RFA for treating other non-cancerous bone lesions such as osteoblastoma, giant cell tumor, enchondroma, eosinophilic granuloma, or bone hemangioma is quite restricted and is confined to a small number of published reports (36). In such instances, RFA should be regarded as a situational instrument that may be contemplated for patients experiencing illness recurrence or those who face a significant likelihood of surgical complications.
Desmoid tumor
Desmoid tumors are rare, non-cancerous, soft tissue neoplasms that originate from musculoaponeurotic structures. They are primarily found in the abdomen, extremities, or abdominal wall. The spontaneous form of desmoid tumors has an estimated prevalence of 2 to 4 cases per million per year. However, these tumors are more frequently observed in people with familial adenomatous polyposis. Surgical excision was the preferred treatment for desmoid tumors, unless there is a high likelihood of major functional loss or morbidity resulting from the procedure (71). Although desmoid tumors are histologically benign, they exhibit local aggressiveness, leading to a high likelihood of local recurrence (usually within 2 years) and making their behavior unpredictable (72). In such instances, alternative non-surgical approaches including radiation therapy or systemic treatments (such as hormones, nonsteroidal anti-inflammatory medications, interferon, and chemotherapy) can be employed to try to achieve local management of the abnormality (73). Minimally invasive methods have been tried to treat desmoid tumors, similar to the ones used for osteoid osteoma and chondroblastoma. Currently, there is limited evidence available regarding the utilization of RFA in this particular area. The existing data consists of a few small case series (74-76), which have demonstrated response rates ranging from 61% to 100%, along with minimal occurrence of complications. CT-guided ablation for desmoid tumors is hindered by the inadequate demarcation of lesion boundaries, as they appear similar in density to muscle tissue. MRI is superior in accurately defining lesions, and as a result, with the advancement of MRI-guided techniques, it may become the preferred imaging method for performing RFA on desmoid tumors.
Malignant bone tumors metastases
The majority of cancer patients experience skeletal metastases, with breast, prostate, kidney, and lung cancers being the principal tumors responsible for metastasis. The axial skeleton, consisting of the spine, head, and pelvis, as well as the hip, ribs, and femur, are the primary sites where this condition is frequently found (1). Skeletal metastases can result in significant morbidity. The condition involves experiencing pain in the bones, suffering from fractures caused by disease, and the impairment of significant nearby structures. The most crucial structures affected are major nerves and the spinal cord. Radiation therapy is the established method used to alleviate bone discomfort caused by the spread of cancer (77). Although radiation is commonly used, its effectiveness could be put to the test since it has early and late pain recurrence rates of up to 40–50%. Furthermore, even in instances of success, the commencement of noticeable alleviation from symptoms may require many weeks and could be restricted in its extent. Certain cancer metastases exhibit inherent resistance to radiotherapy (5), whereas irradiation-sensitive lesions have a maximum dose threshold beyond which additional radiation doses are deemed intolerable, therefore preventing the indefinite use of radiotherapy. In many circumstances, a minimally invasive therapeutic strategy is required due to several factors. Among the numerous possibilities, RFA is considered the primary and most frequently recognized option. Below, we will examine the primary indications in different clinical scenarios for RFA.
Bone metastases causing significant pain
The most widely recognized reason for ablating MSK metastases is to alleviate painful skeletal metastatic illness. Callstrom et al. originally reported the application of image-guided RFA for treating these lesions in 2002 (78). Subsequent clinical trials conducted at multiple centers have provided evidence that RFA is both safe and effective during a follow-up period of 3–6 months (79-81). This is particularly true when RFA is used on lesions measuring up to 3 cm, primarily consisting of osteolytic or a combination of osteolytic and osteoblastic features (11,82). Otherwise, RFA is relatively ineffective in treating densely mineralized tumors (18). Furthermore, a matched cohort trial conducted by Di Staso et al. (83) shown that the combination of RFA and radiotherapy is more effective than radiation alone in managing pain associated with osteolytic bone metastases. Regarding spinal metastases, RFA is recommended as part of the treatment plan for metastases that are asymptomatic, causing pain, or resulting in fractures (without instability or compression of the spinal cord). Additionally, RFA is suitable for patients with a life expectancy of more than 6 months, a good performance status, and only a few visceral metastases (84).
Local management of oligometastatic disease
Recently, there has been an increase in the use of aggressive treatment for patients with limited metastatic disease in the field of oncology (85). Due to the high occurrence of prostate and breast cancer, as well as the natural resistance of certain diseases (such as melanoma, kidney cancer, and non-small cell lung cancer) to radiotherapy, there is a growing interest in utilizing minimally invasive ablation techniques to treat oligometastases. Several studies have been done to evaluate the efficacy of RFA in achieving durable local tumor control in appropriately selected patients with limited metastases of kidney, breast, and prostate cancer at MSK (86-88). The investigations indicated that local control rates ranged from 67% to 93% throughout follow-up periods of 8 to 23 months. The complication rates were found to be between 0% and 9%. These findings demonstrate that RFA is a viable method that can be employed to delay the need of systemic medications, which may have adverse consequences, in these individuals.
Constraints and follow-up of bone metastases
Although RFA has been shown to be useful, it is important to acknowledge certain limitations when using it to bone metastases. Firstly, the absence of water in highly sclerotic lesions results in a significant increase in impedance, which may hinder the effectiveness of RFA in completely destroying the lesion. However, the bone’s stability may be impaired following ablation, which may necessitate the use of both RFA and cementoplasty in certain circumstances (89). Imaging follow-up in patients treated for painful metastases often varies and is necessary either for evaluating the overall disease status or for excluding post-ablation problems. Nevertheless, for patients undergoing treatment to manage local tumors, it is recommended to have regular and consistent imaging follow-up. This usually involves imaging at 3-, 6-, and 12-month following ablation, and then every 6–12 months thereafter (5).
RFA in MSK tumors contraindications and complications
Patients with non-compatible MRI pacemakers or metallic implants should not undergo RFA to prevent adverse physiological consequences. While RFA is a procedure that involves minimum invasion, it is not without possible consequences. Hemorrhaging or harm to nearby neural tissues may occur during the insertion of a needle and can be prevented by possessing accurate anatomical understanding and careful planning of the approach. Soft-tissue and skin burns may arise from the insertion cannula and the grounding pads, potentially leading to posterior infection, necrosis, and abscess formation. Ultimately, in lesions that are of considerable size or placed in joints that bear weight, there is a potential for bone demineralization, pathological fracture, and cartilage impairment (90). It is advisable to refrain from engaging in intense physical activity for approximately 3 months following ablation in such situations.
Microwave thermal ablation (MWA)
Procedure
MWA involves using an antenna to deliver electromagnetic energy at frequencies about 900 and 2,450 MHz to target tissue. This process causes molecular agitation, leading to tissue heating and coagulative necrosis. Microwaves can achieve efficient coagulation at a faster rate compared to other ablation methods (11,35,44). MWA is believed to be less affected by changing tissue impedance and perfusion-related tissue cooling, resulting in higher temperatures within the tumor, a bigger and more consistent ablation area, and more effective ablation with just one antenna.
Advantages
MWA is successful in managing osteoblastic lesions and eliminates the need for grounding pads, reducing the danger of skin burn (1,11,18,91) (Figure 4). MWA has been successful used in treating osteoid osteomas. Additionally, it can be utilized for neurolysis as well (10). MWA energy may penetrate all types of biological tissue and allows for quicker and more extensive ablations compared to RFA, reaching up to 8 cm when multiple antennas are utilized concurrently (11,18,44,93).
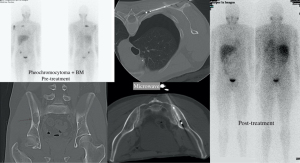
Disadvantages
While hypoattenuating ablated tissue can be seen on CT scans, the boundaries of the ablation zone are not clearly defined, which is a challenge for spinal MWA procedures (91). Moreover, the intact bone cortex does not impede the propagation of microwave energy, which can lead to overheating due to high-power energy deposition (18). Another drawback of MWA is the limited knowledge on its effectiveness for treating bone metastases due to its recent introduction as a therapy (18,93,94).
Cryoablation (CA)
Procedure
Thermal CA involves using intense cold to eliminate tumors. For CA, small 17-gauge gas-driven cryoprobes are employed and guided percutaneously by CT or MR imaging. The cryoprobe rapidly expands high-pressure argon gas, causing a quick temperature drop below 183 ℃ through the Joule-Thompson effect, which takes around 10 minutes to occur. Rapid decompression of helium gas causes the temperature to increase to +33 ℃, leading to active thawing, which takes roughly 5–8 minutes to occur (11,18,34,44,95). Repeating freeze-thaw cycles is crucial for successful cryopreservation. Initially, ice crystals form outside the cells during freezing. Subsequently, as the cells thaw, water moves into the cells due to osmotic differences, resulting in intracellular ice crystal formation during the second freezing phase. This process ultimately causes membrane damage and cell death (18,44). Cell damage increases with the prolonged duration of the thawing phase. The ice ball’s spherical margin should extend around 3–5 mm beyond the tumor margin to guarantee sufficient coverage (10,11,18,44).
Indications
CA is the treatment of choice for bone metastases with soft tissue extension (Figures 5,6), big lesions affecting the posterior spinal elements, and osteoblastic lesions (91,93,95). Other benign tumors that may be treated with CA include extra-abdominal desmoid tumors, osteoid osteoma, osteoblastoma, chondroblastoma, aneurysmal bone cysts, or Morton’s neuroma (35). Additionally, it can be utilized for neurolysis as well (10).
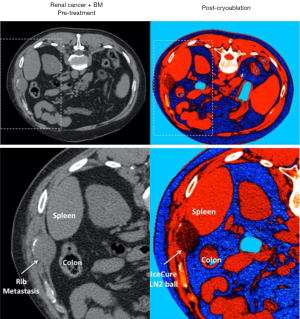
Advantages
Accurate control of the ablation area is achieved by clear visualization of the hypoattenuating ice ball on CT scans. Moreover, several probes can be engaged at the same time to mold the ice ball based on tumor shape, allowing for the treatment of extensive bone metastases (11,18,44). Finally, ice ball possesses inherent anesthetic characteristics, making operations less unpleasant compared to heat-based approaches.
Disadvantages
CA is costly and time-consuming, taking about 25–30 minutes. Additionally, it is noted that CA can cause thermal damage to the spinal cord and nerve roots, particularly because the bone cortex does not prevent the expansion of the ice ball (11,18,34).
High intensity focused ultrasound (HIFU) thermal ablation
Introduction
The effectiveness of ultrasound in heating tissues was initially acknowledged during World War II, when high-intensity ultrasound waves were utilized for submarine navigation, resulting in the observation that these waves caused warmth and death in fish (96). In subsequent years, researchers directed ultrasonic waves towards bodily tissues as a substitute for ablative techniques based on that discovery (97). Over time, further study has shown that ultrasound is an excellent and non-invasive method for ablating soft tissues. Ultrasound has significant advantages compared to other treatments, including the ability to treat deeper tissues, enhance focus on target tissues with its short wavelengths, and provide exact control over the shape and placement of energy deposition (98).
Physical principles behind HIFU
The basis of HIFU ablation consists of the application of an US beam that passes through the overlying skin and tissues and focus on a single point or region, that may be deep within the tissues, causing coagulative necrosis (11,18,35,99). Focused ultrasound therapeutic action is characterized by two mechanisms. The first is a thermal effect in which the absorption of ultrasound energy in a focal point develops a rapid temperature to rise in the exposed tissue to more than 60°, thus inducing irreversible cell death (18,100). The second mechanism, known as acoustic cavitation, is a mechanical effect involving microscopic streaming fluid-jets induced by high pressure, temperature and stress mechanisms, that can lead to cell apoptosis (101). Although the US beam focuses on a small volume of about 1–3 mm in diameter and 10 mm in length, the ablation area can reach an upper size limit of approximately 3–4 cm in diameter. When the target lesion is ablated, a very sharp perimeter between totally disrupted cells and normal tissue is created, measuring no more than 50 µm (100,102). An important factor to consider when planning HIFU ablation is the presence of air or potential US beam-reflecting structures such as scars, ligaments and tendons. If these structures are interposed between the beam origin and target lesion; they can obstruct wave transmission and induce thermal damage to the structures located along the beam trajectory, resulting in possible skin burns (103).
HIFU ablation equipment
Ultrasound beam delivery system
The primary element of HIFU equipment is the piezoelectric ultrasonic transducer responsible for delivering the therapeutic beam. Various types of transducers include concave focusing transducers with fixed aperture and focal length, phased array transducers with numerous piston transducers, and flat transducer/fully populated phased array. The placement of transducers during the ablation treatment is determined by the level of accessibility to ultrasound of the target tissue or region. When the organ is easily reached, such as the kidney, an external or extracorporeal transducer is utilized to direct the ultrasound beam through an acoustic window on the skin. These external devices utilize a wide aperture and long focal length to reduce the acoustic strength at the wave’s entry point in order to prevent skin burns (99). When the target lesion is located in a deeper organ, the transducer may need to be inserted into the body and positioned close to the targeted region. In such scenarios, transducers often function at elevated frequencies and reduced power levels to be effective at shorter distances (100). For instance, in cases of prostate cancer, transrectal transducers are utilized. Novel interstitial devices based on percutaneous, endoscopic, laparoscopic, or catheter-based ultrasound devices are under development for treating malignancies such esophageal or biliary duct tumors (104,105).
Imaging guidance modalities
Real-time monitoring of the procedure is essential for the safety and efficiency of the therapy. Another important aspect of this ablation system is the imaging modalities employed for guidance. Two primary imaging approaches have been utilized to direct HIFU:
- MRI: is highly effective for visualizing target lesions and planning treatment due to its superior anatomical resolution and sensitivity for detecting tumors. Unlike US, this method is not restricted by fat tissue, making it beneficial for treating obese individuals. Additionally, it allows for magnetic resonance (MR) thermometry, which includes the live tracking of the thermal dosage administered to tissues that can be overlaid onto an anatomical image (106-108).
- US: uses ultrasound imaging to provide real-time verification of the acoustic window by utilizing the same energy for guidance as for therapy. US-guidance is effective for pre-procedural placement of the target tumor but not for evaluating therapeutic boundaries during the procedure because to the limited visualization of the ablated region in standard B-mode images (99).
Ablation procedure
The objective of the HIFU ablation method is to administer sufficient energy to the specified area to guarantee total elimination of the lesion together with a 5 mm safety margin (108). US-guided focused ultrasound (USgFUS) is effective for superficial soft tissue lesions in MSK cases but has problems in accurately imaging bone lesions. As a result, MR-guided focused ultrasound (MRgFUS) has gained increased popularity and gathered more supporting data. Due to its complexity and additional processes, only the MRgFUS technique is discussed now (109). The operation necessitates sedation and analgesia, which can vary from local anesthesia with conscious sedation to general anesthesia based on the patient’s characteristics. Prior to patient positioning, T1- and T2-weighted MR sequences are obtained with or without fat saturation to locate the target lesion and strategize an approach that avoids important structures and structures that reflect ultrasound. The patient is placed on the MRgFUS table with the target tumor positioned directly above the ultrasound transducer. When using an extracorporeal transducer throughout the process, it is essential for it to be in direct contact with the patient’s skin and for the surfaces to be kept moist with degassed water. The acquired images are sent to the MRgFUS workstation for manual segmentation. A zone of interest is outlined around the target area, and sections to be avoided are contoured. The treatment involves a sequence of energy depositions referred to as sonication. Prior to commencing therapeutic sonication, a low-energy sonication must be conducted to confirm the accurate positioning of the target area. Subsequently, full-energy sonication is conducted for 20 to 30 seconds until the entire target area is ablated. MR-thermometry with real-time temperature maps is utilized to guarantee that the tumor reaches the crucial ablation temperature of 65 ℃ during the complete process. The frequency of therapeutic ultrasound is determined by the depth of the target lesion and the desired heating rate for treatment. Higher frequencies, such as 8 MHz, exhibit shallower penetration depths, whereas lower frequencies, like 0.5 MHz, have greater penetration depths. Frequency around 1 MHz has been found to be the most effective for heat deposition (99). For cases needing a larger target volume for ablation, the MRgFUS transducers are incrementally shifted and activated at each point until a continuous area of ablated tissue is achieved. After receiving local anesthesia, patients should have their skin examined for burns or thermal damage, vital signs monitored for 2 hours, be given pain or nausea medication as needed, and receive morphine infusion for 24 hours post-treatment. Conversely, individuals who receive general anesthesia typically require a 24-hour hospital stay following the procedure.
The following table summarizes the main advantages and disadvantages of HIFU compared to other ablation techniques (Table 2).
Table 2
Advantages of HIFU ablation | Disadvantages of HIFU ablation |
---|---|
• Decreased toxicity associated with ablation | • Responsive to patient motion |
• Non-invasive approach is typically used | • Heating nearby structures is possible |
• Causes slight discomfort | • Limited effectiveness for tumors within 1 cm of the skin surface and neural structures. Infeasibility of treating spinal tumors (11,18,35) |
• Typically necessitates reduced anesthesia | • If guided by MRI, the treatment may be lengthy (18) |
• Absence of ionizing radiation exposure | • Claustrophobic patients may decline MR-guided therapy |
• Outstanding consistency with no dosage restrictions | • Ultrasound interference caused by reflecting structures such as metallic implants and unintended bone in the path of the ultrasound beam (11,18) |
• Accurate energy distribution to a specific location without impacting the integrity of the skin | • Only bone lesions that are superficial enough can be treated since the cortical bone hinders the penetration of the ultrasound beam (18,35) |
• Minimal impact on adjacent tissues in terms of negative effects | |
• Feasibility of real-time monitoring and visualization of the process (11,35) | |
• Continuous temperature mapping (11) |
HIFU, high-intensity focused ultrasound; MRI, magnetic resonance imaging; MR, magnetic resonance.
The incidence of problems related to HIFU ablation of MSK lesions is minimal. The most commonly reported consequences are mild local pain, skin burns, tissue and peripheral nerve damage, bone fractures, and secondary infections (82,109-111).
Indications
Several HIFU applications have been documented for treating MSK diseases, including alleviating pain from metastatic tumors, treating initial bone malignancies, and addressing some benign tumors like osteoid osteoma or desmoid tumor.
Pain palliation of metastatic tumors
The versatility and effectiveness of minimally invasive ablation methods in managing painful bone metastases are well-established (82). Preliminary clinical investigations (phase I/II trials) on MRgFUS have shown high safety and response rates, ranging from 72% to 100% (82,112-115). While the exact mechanism of action is still uncertain, these studies emphasize that tumor debulking, along with periosteal denervation, may have a clinically substantial impact on symptom alleviation. In 2013, Hurwitz et al. (111) conducted the sole prospective, double-blinded, phase II clinical investigation on the application of MRgFUS for alleviating painful bone metastases. The therapy arm demonstrated a 3-month response rate of 64%, compared to 20% in the placebo arm, along with a 47% decrease in the intake of pain-relief medication. The overall safety profile was favorable. Considering these findings, MRgFUS is a feasible, secure, and efficient treatment choice for painful bone metastases in patients for whom radiation therapy has been unsuccessful or who are ineligible or decline this treatment.
Primary bone malignant tumors treatment
Radical surgery has traditionally been the preferred treatment for primary bone cancers. In recent decades, there has been a gradual transition towards preserving limbs with full local function, resulting in the adoption of this technique as a common practice for primary bone cancers (116). In 2010, two trials assessed the effectiveness and safety of HIFU ablation combined with chemotherapy for primary bone lesions, despite the general agreement on the limited efficacy of US energy for therapeutic ablation in this context (117,118). The studies indicated complete response rates of 85–86% following one or more treatment sessions, along with relief in tumor-related discomfort and a recurrence rate of 7%. These studies indicate that combining HIFU with chemotherapy could be a safe and viable treatment option for patients with malignant bone tumors.
Treatment of benign tumors
Percutaneous RFA is the primary treatment for painful osteoid osteoma due to its high success rates and minimal complication rates (100). Nevertheless, this process carries certain hazards, particularly in children, due to the utilization of needles and radiation. MRgFUS is emerging as a potential alternative to traditional ablation procedures for these reasons. Various studies have shown MRgFUS to be highly successful, with complete response rates reaching up to 97%, and safe, with few to no problems (103,119-121). Therefore, MRgFUS can be seen as a viable substitute for RFA in treating osteoid osteomas.
Desmoid tumors are a form of benign MSK growths that could benefit from HIFU ablation. The available evidence from a small number of studies indicates that MRgFUS could be a viable and safe option for treating extra-abdominal desmoid tumors by either eliminating viable tumors or controlling tumor growth over time with repeated treatments (122-124).
Other applications
In recent years, numerous potential uses for HIFU have surfaced and necessitate additional research. A study in 2015 illustrated HIFU as a possible method for blocking blood arteries in many disorders, such as controlling bleeding in arteriovenous malformations or reducing solid tumors by interrupting their blood flow (125). A recent feasibility study assessed the effectiveness of HIFU in treating abscesses, particularly those produced by challenging-to-treat methicillin-resistant Staphylococcus aureus (MRSA). The study utilized HIFU at two focal temperatures, 52 and 64 ℃, to treat MRSA-induced abscesses in mice. Following the HIFU ablation at 64 ℃, there was a significant decrease in bacterial load and external abscess size at days 4 and 10, respectively (126).
Treatment strategies for the ablation of MSK tumors: indications and patient selection
Patients with bone metastases should have treatment programs determined by a multidisciplinary team (91). The “Cardiovascular and Interventional Radiological Society of Europe (CIRSE). Quality Improvement guidelines for bone tumor management” states that interventional treatment strategies might be either palliative or curative.
Palliative treatments
Ablation procedures are recommended for the majority of patients with bone metastases to address skeletal associated problems (1). The objective is not total tumor elimination but rather pain relief, avoidance of pathological fractures, and/or reduction in tumor size (particularly for spine tumors extending into the spinal canal). However, tumors located less than 1 cm from important structures such as the spinal cord or big blood arteries constitute a relative contraindication if these areas cannot be safeguarded (35). These treatments are recommended for cases of persistent pain that do not improve with maximum radiation therapy, when radiation therapy is not suitable, or when systemic therapies and analgesics are not effective (91,93). To qualify for palliative treatment, patients must have either one painful lesion or a few painful lesions that are supported by imaging results indicating a pain level of 4 or above on a pain scale observed during a physical examination (5). Palliative care can be administered for bone metastases that spread to nearby soft tissues or spinal tumors that rapidly invade the spinal canal.
Curative treatments
Curative treatment refers to the total and conclusive ablation of a malignancy. Curative therapy may be recommended for oligometastatic patients under 60 years old who have limited bone disease, namely less than 3 possibly curable bone metastases, each with a maximum size of 3 cm. To ensure successful local management, the safety margins should exceed the lesion by at least 0.5–1 cm (35). Additionally, it may be recommended for patients with a slowly progressing disease and those with long-term stable systemic metastasis and one or a few bone metastases that do not respond to traditional systemic treatments (i.e., oligo-progression) (35,91).
Thermal protection and monitoring for avoiding complications
Thermal ablation is typically regarded as safe. Common problems sometimes involve the inadvertent removal of adjacent non-target organs such the spinal cord, nerves, articular cartilage, arteries, skin, or essential organs (127,128). Passive thermal protection involves monitoring patient input, real-time temperature monitoring during procedures using thermocouples placed near vulnerable structures, and neurophysiological monitoring. It is advisable to use active thermal protection when the temperature is between 45° and −10°. This can be achieved through temperature alteration using techniques such as pneumodissection with carbon dioxide insufflation, hydrodissection with warm or cold liquid, or by adding warm saline to the skin surface (11,18).
If the patient experiences sensory-neural impairments during conscious sedation, the treatment should be halted and active thermo-protective methods such CO2 insufflation and cooling/warming with fluid should be employed. Hydrodissection involves using non-ionic solutions like 5% dextrose-in-water to move the at-risk structure by changing the temperature of the surroundings. Approach intra-articular lesions carefully to reduce the risk of damaging the subchondral bone plate and the articular cartilage above it. This can be accomplished by injecting dextrose 5% in water or carbon dioxide into the joint. Neurophysiological monitoring (129) and nerve electro-stimulation (130) during thermal ablation can detect early signs of reduced motor and somatosensory evoked potential amplitudes indicating impending neurologic damage. Skin damage can occur as a potential complication of percutaneous thermal ablation. Warm saline applied to the skin during CA helps reduce skin injury, whereas grounding pads lower the risk of skin harm in unipolar RFA systems. Thermal ablation can be coupled with cementoplasty to reduce the risk of pathologic fracture in bone metastases.
Percutaneous cementoplasty
Cementoplasty is a fundamental procedure in percutaneous bone consolidation procedures (131). The technique was initially introduced by Galibert in 1987 (132) as a treatment for a highly aggressive cervical hemangioma. Since then, its application has been expanded to include many types of osteoplasties, particularly for the management of bone fractures, irrespective of their underlying cause. Originally, the procedure was restricted to vertebrae (vertebroplasty) (133), but its applications quickly expanded to include areas outside of the spine. The purpose of cementation is to strengthen the diseased bone by stabilizing both large and small fractures (134). Moreover, acrylic cement is considered cytotoxic because to its heat reaction during polymerization, which can reach temperatures of 80 ℃ and could cause coagulation and destruction of tumor cells and nerve terminals (135-138). Nevertheless, the exact mechanism of action for cementoplasty remains elusive, resulting in its usage being primarily palliative. Cement is known for its high resistance to compressive pressures, however it is somewhat less resistant to torsional forces (139). Consequently, it has primarily been utilized to consolidate spinal and acetabular bone defects (133,140,141). Long bones are more susceptible to torsional pressures, which can result in inferior outcomes in these areas (142). In these instances, cementoplasty may alleviate the pain, but it will not offer efficient and long-lasting consolidation and stabilization (11). Poly-methyl-methacrylate (PMMA) is the predominant acrylic cement utilized, however alternative options such as composite or calcium phosphate cements are also viable (143).
Technique
The method involves injecting PMMA into a fractured or weakened vertebra or another bone section affected by diseased tissue (10,35,44). The procedure is often conducted with the administration of local anesthesia (18), and may or may not involve the use of neuroleptanalgesia. Fluoroscopy or CT imaging may be utilized during the procedure. The cement is administered as paste substance and it undergoes solidification within a time frame of roughly 20–30 minutes (35). An additional option, the balloon kyphoplasty, may enhance the efficacy of tumor filling in instances of spinal metastases (11). Hemodynamic monitoring is necessary during the treatment due to the potential of acrylic cement injections to induce transient decreases in arterial pressure (45). The procedure is conducted under sterile conditions, typically with at least two operators, a principal operator and an assistant. Immunocompromised individuals must utilize a prophylactic broad-spectrum antibiotic, specifically cefazolin 1 g (143,144). The initial access site is typically selected using CT, and once the needle is in the most favorable place, guiding can be transitioned to fluoroscopy or maintained with CT. A biplane is a type of aircraft with two sets of wings, one above the other. Additionally, when it comes to medical procedures, fluoroscopy or dual guiding using a C-arm can also be considered as an alternative option. Local anesthesia is typically delivered in the subcutaneous tissues and in the periosteum of the specific bone being targeted. The needles commonly employed for cement injection typically have a gauge size ranging from 10 to 14 G in nearly all processes (34). Aseptic surgical mallets are typically employed to facilitate cortical perforation. When the needle is in the ideal location, the acrylic cement is combined with tantalum (to enhance radio-opacity). During the initial 30–50 seconds after mixing, the glue has a thin consistency. However, it gradually thickens and becomes pasty. It is crucial to do the injection during this pasty polymerization phase to avoid the migration of the glue to distant veins. A pressure syringe is a device used to administer fluids or medications into the body by applying force to push the substance via a narrow tube or needle, to facilitate the introduction of the thick blend. At this juncture, it is imperative to expedite the injection of the cement due to its tendency to thicken after 3 minutes, which hampers subsequent injection. Fluoroscopy (Figure 7A-7E) should be used to closely monitor and regulate all cement injection procedures. CT guidance is more effective in detecting cement leakage because to its greater spatial resolution (145,146). Several authors employ the technique of simultaneous injection through numerous bone trocars (147). This method offers efficient pain alleviation, however, it is not associated with the quantity of cement administered. This phenomenon is particularly significant in the context of metastasis, as a little 1.5 mL of cement typically suffices to significantly alleviate discomfort (148). Indeed, throughout the process of vertebroplasty, the injection is halted upon the detection of epidural or paravertebral opacification. For extraspinal cementoplasty, there is no universally agreed upon amount of cement to use. Typically, the cement is filled as much as feasible in both the lesion and the surrounding cancellous bone to achieve the best possible bone consolidation (139,149). The influence of lesion filling extent on mechanical outcomes in clinical practice remains uncertain. Therefore, it is necessary to always adjust optimal filling taking into account the possibility of leakage (148). If imaging is unable to accurately detect the bone tumor, a needle core biopsy can be conducted concurrently utilizing a coaxial needle. Percutaneous bone consolidation can be integrated with additional interventional therapeutic radiology techniques such as embolization, RF, or thermal ablation. These strategies facilitate the dispersion of PMMA by causing tumor and tissue destruction, perhaps resulting in a synergistic impact (150-154). However, certain authors suggest that it may be linked to reduced rates of leakage (155). Nevertheless, accurately evaluating the actual advantages of ablation and cementoplasty is a challenge. Garnon et al. (148) states that thermal ablation effectively reduces discomfort resulting from the infiltration of the surrounding muscle in cases of significant osteolytic metastases. Cementoplasty can be used in conjunction with surgical fixation or decompression, particularly in cases with spinal lesions. In such situations, combining vertebroplasty with laminectomy and posterior fixation can be beneficial in avoiding the need for anterior osteosynthesis, therefore lowering morbidity (155). The limited resistance of PMMA to torsional stress renders this technique unsuitable as a standalone treatment for metaphyseal or diaphyseal lesions that have a high likelihood of fractures (156-158). In such instances, implant devices can be utilized to secure the location, while cement enhances the fixation of the device.
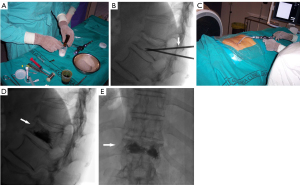
Advantages
Percutaneous cementoplasty demonstrates immediate and enduring efficacy, in contrast to the delayed efficacy shown with irradiation. Cementoplasty not only has an analgesic effect but also promotes bone consolidation (133,140,143). In addition, percutaneous cementoplasty is employed as a method of pain management for patients suffering from bone failure or osteoporosis, conditions commonly observed in oncology patients due to factors such as pathological fractures, prolonged steroid use, and advanced age. Preventing pathological fractures enables the preservation of limb movement, leading to enhanced performance status and quality of life for patients (144,148). Percutaneous cementoplasty does not require always general anesthesia, making it suitable for extremely delicate patients who are deemed unfit for surgery due to the associated risks. The incidence of blood loss and surgical trauma is reduced compared to traditional surgery, resulting in decreased infection rates and associated comorbidities. In addition, this technique also decreases the duration of hospitalization and the likelihood of thromboembolic consequences (148,159). Finally, PMMA, being an affordable and readily available material, significantly reduces the cost of this operation in comparison to surgery (136).
Indications
Percutaneous cementoplasty might be employed to treat painful fractures caused by osteoporosis or osteonecrosis, conditions commonly observed in oncology patients. Other indication for percutaneous cementoplasty is a painful bone tumor, particularly metastasis and myeloma, especially in cases where there is a significant danger of compression fracture (34,35,144). Treatments are available for benign bone lesions, such as symptomatic vertebral tumors such aggressive hemangiomas, giant cell tumors, and aneurysmal bone cysts (132,160). Spinal lesions have been documented in various scientific publications (133,134,136,138,144,160,161). Additionally, there are other bone lesions located outside the spine that have been less frequently reported but may still be treatable. These include lytic lesions of the acetabulum (140,141,162), sacrum, superior and inferior pubic rami, ischial tuberosities, mandible and maxilla, as well as long bones in the metaphysis or epiphyses (136,139,142,148,155,156,158,163,164). There is a scarcity of studies providing results on the long bone diaphysis, which some authors advise against due to the cement’s limited ability to withstand torsional forces. Deschamps et al. (158) proposed that percutaneous cementoplasty could be a viable method for treating proximal femur lesions with cortical involvement less than 30 mm or a prior fracture of the lesser trochanter. Mirels et al. (165) propose that for lengthy bone lesions, a preventive fixation should be considered if the overall score is 9 or higher. This procedure may involve cementoplasty or not. Lesions meeting this criteria should be classed as imminent fractures. Cementoplasty is a suitable treatment for symptomatic osteoblastic metastases when the afflicted bone is not highly thick and there are fissures present. When there is a significant osteoblastic reaction, filling the bone with cement may result in leaking around the bone (155).
Contraindications
Cementoplasty is mostly a palliative technique that should only be regarded as an analgesic and consolidative treatment (140,141,148). Similar to other percutaneous procedures, there are just a few contraindications (144):
- Coagulopathy and hemorrhagic diathesis (if unable to be corrected).
- Infection: discitis, osteomyelitis, or ongoing systemic infection.
- Hypersensitivity to opacification chemicals or bone cement.
- Spinal fracture with an unstable condition. The absence of stability, despite the rupture of the posterior wall, does not necessarily prevent the procedure. However, it is important to exercise attention to prevent the leakage of cement near the nerves (166).
- Spinal cord compression is not an absolute reason to exclude someone from treatment. However, it is recommended to combine it with steroids, surgery, or radiation therapy. Exercise cautious when administering injections to prevent spinal cord compression (136).
While diffuse metastases does not provide a direct obstacle, the existence of over 5 vertebral lesions is considered a relative contraindication (144).
Complications
Oncologic lesions have a higher incidence of complications compared to osteoporotic fractures. The suggested threshold for complications at a specialized tertiary center is 10% for malignant cases and 2% for osteoporotic fractures (167). The most common problem is cement leakage, which occurs when cement seeps into nearby structures through flaws in the cortical bone or through venous channels (34,44). To minimize the risk of cement leakage, it is recommended to use a transpedicular approach (18). Subsequent radiographs reveal cement leakage in a range of 30% to 72.5% of the procedures, with a maximum of 90% observed on CT scans (35,167,168). The extent of leakage is contingent upon the anatomical structures that are impacted. An epidural leak with spinal cord compression is the most severe complication, potentially necessitating immediate decompression surgery. Penetration into the paravertebral veins can lead to pulmonary cement embolism (169), a condition that can occur in around 10% of patients, with the majority being asymptomatic (152). The occurrence of paradoxical cerebral cement embolism has been documented (170). Radiculopathy can be caused by neural foramina leakage. Disc leaks typically do not show any symptoms, but they can potentially raise the likelihood of nearby bone collapse (166). To prevent this issue, it is advisable to closely monitor the cement injection process and utilize a reduced volume of cement (171). The incidence of infection in the procedures is less than 1% (172). The implementation of rigorous sterility measures and the administration of prophylactic antibiotics can effectively prevent this problem. Pain and fever may occur temporarily within the initial 24-hour period (166). The level of discomfort experienced is typically directly proportional to the amount of cement injected (171) and may be attributed to the exothermic reaction of PMMA. As previously stated, there is a heightened risk of subsequent fracture when treating lengthy bones. In their study, Garnon et al. (148) observed secondary fractures in 16 cases, which accounted for 8% of the total cases, following cementoplasty. Patients who are not bedridden and have a sufficiently long life expectancy should always be evaluated for surgical intervention. The incidence of periprocedural fracture, such as rib fractures in vertebroplasty, is less than 1% (143). Additional complexities have been delineated.
Local hemorrhage and hematoma in the soft tissue is an infrequently reported consequence, often regarded as trivial. Acute hypersensitivity to acrylic cement and other filling materials may manifest as an allergic reaction. Physicians should be cognizant of any allergic reactions, such as coughing and bronchospasm, that may occur during the procedure (173). An abrupt rise in the concentration of cancer cells in the bloodstream has been reported shortly after injection (174). Ultimately, percutaneous cementoplasty is a highly effective treatment. Undoubtedly, in a recent analysis, 21 out of 24 trials documented a decrease beyond 2 in the pain score (which is regarded as the critical point for statistical significance). A significant number of series have reported pain relief in up to 83% of the treatments (141,148). Successful vertebroplasty relies on several crucial factors, including appropriate patient selection, accurate needle positioning, precise timing of cement injection, meticulous fluoroscopic guidance, and the expertise of the operator (136,143,144,148,159,164,166,175,176).
Conclusions
In summary, percutaneous image-guided minimally invasive interventional therapy represents an excellent approach for treating MSK tumors. Over the past decade, significant progress has been made in this topic, leading to its widespread acceptance in clinical practice. CT imaging remains the favored method due to its availability and ability to visualize tumor and surrounding structures. These interventional techniques, including ethanol injection, RFA, microwave ablation, CA, and MR-guided HIFU, have been effectively employed. Combining ablation with other interventions, such as cementation or embolization, can enhance local tumor control. This article provides an overview of the essential components of minimally invasive interventional guided imaging therapy for MSK tumors.
Acknowledgments
Funding: None.
Footnote
Provenance and Peer Review: With the arrangement by the Guest Editors and the editorial office, this article has been reviewed by external peers.
Conflicts of Interest: All authors have completed the ICMJE uniform disclosure form (available at https://qims.amegroups.com/article/view/10.21037/qims-24-452/coif). The special issue “Advances in Diagnostic Musculoskeletal Imaging and Image-guided Therapy” was commissioned by the editorial office without any funding or sponsorship. X.T. and J.M.V. served as the unpaid Guest Editors of the issue. The authors have no other conflicts of interest to declare.
Ethical Statement: The authors are accountable for all aspects of the work in ensuring that questions related to the accuracy or integrity of any part of the work are appropriately investigated and resolved.
Open Access Statement: This is an Open Access article distributed in accordance with the Creative Commons Attribution-NonCommercial-NoDerivs 4.0 International License (CC BY-NC-ND 4.0), which permits the non-commercial replication and distribution of the article with the strict proviso that no changes or edits are made and the original work is properly cited (including links to both the formal publication through the relevant DOI and the license). See: https://creativecommons.org/licenses/by-nc-nd/4.0/.
References
- Coleman RE. Clinical features of metastatic bone disease and risk of skeletal morbidity. Clin Cancer Res 2006;12:6243s-9s. [Crossref] [PubMed]
- Mundy GR. Metastasis to bone: causes, consequences and therapeutic opportunities. Nat Rev Cancer 2002;2:584-93. [Crossref] [PubMed]
- Janjan N. Bone metastases: approaches to management. Semin Oncol 2001;28:28-34. [Crossref] [PubMed]
- Kurup AN, Callstrom MR. Increasing Role of Image-Guided Ablation in the Treatment of Musculoskeletal Tumors. Cancer J 2016;22:401-10. [Crossref] [PubMed]
- Kurup AN, Morris JM, Callstrom MR. Ablation of Musculoskeletal Metastases. AJR Am J Roentgenol 2017;209:713-21. [Crossref] [PubMed]
- Filippiadis DK, Tutton S, Kelekis A. Percutaneous bone lesion ablation. Radiol Med 2014;119:462-9. [Crossref] [PubMed]
- Gangi A, Buy X. Percutaneous bone tumor management. Semin Intervent Radiol 2010;27:124-36. [Crossref] [PubMed]
- Leyendecker JR, Dodd GD 3rd. Minimally invasive techniques for the treatment of liver tumors. Semin Liver Dis 2001;21:283-91. [Crossref] [PubMed]
- Moser T, Buy X, Goyault G, Tok C, Irani F, Gangi A. Image-guided ablation of bone tumors: review of current techniques. J Radiol 2008;89:461-71. [Crossref] [PubMed]
- Tomasian A, Filippiadis DK, Tutton S, Deschamps F, Cazzato RL, Prologo JD, Kelekis A, Levy J, Gangi A, Garnon J, Jennings JW. Comprehensive Palliative Musculoskeletal Interventional Radiology Care for Patients with Cancer. Radiographics 2022;42:1654-69. [Crossref] [PubMed]
- Tomasian A, Jennings JW. Bone Metastases: State of the Art in Minimally Invasive Interventional Oncology. Radiographics 2021;41:1475-92. [Crossref] [PubMed]
- Goyal M, Mishra NK, Sharma A, Gaikwad SB, Mohanty BK, Sharma S. Alcohol ablation of symptomatic vertebral hemangiomas. AJNR Am J Neuroradiol 1999;20:1091-6.
- Yang ZY, Zhang LJ, Chen ZX, Hu HY. Hemangioma of the vertebral column. A report on twenty-three patients with special reference to functional recovery after radiation therapy. Acta Radiol Oncol 1985;24:129-32. [Crossref] [PubMed]
- Fox MW, Onofrio BM. The natural history and management of symptomatic and asymptomatic vertebral hemangiomas. J Neurosurg 1993;78:36-45. [Crossref] [PubMed]
- Gangi A, Kastler B, Klinkert A, Dietemann JL. Injection of alcohol into bone metastases under CT guidance. J Comput Assist Tomogr 1994;18:932-5. [Crossref] [PubMed]
- Nair RT, vanSonnenberg E, Shankar S, Morrison PR, Gill RR, Tuncali K, Silverman SG. Visceral and soft-tissue tumors: radiofrequency and alcohol ablation for pain relief--initial experience. Radiology 2008;248:1067-76. [Crossref] [PubMed]
- Akhlaghpoor S, Tomasian A, Arjmand Shabestari A, Ebrahimi M, Alinaghizadeh MR. Percutaneous osteoid osteoma treatment with combination of radiofrequency and alcohol ablation. Clin Radiol 2007;62:268-73. [Crossref] [PubMed]
- Tomasian A, Cazzato RL, Sharma K, Gangi A, Jennings JW. Benign Bone Tumors: State of the Art in Minimally Invasive Percutaneous Interventions. Radiographics 2023;43:e220041. [Crossref] [PubMed]
- Heiss JD, Doppman JL, Oldfield EH. Brief report: relief of spinal cord compression from vertebral hemangioma by intralesional injection of absolute ethanol. N Engl J Med 1994;331:508-11. [Crossref] [PubMed]
- Doppman JL, Oldfield EH, Heiss JD. Symptomatic vertebral hemangiomas: treatment by means of direct intralesional injection of ethanol. Radiology 2000;214:341-8. [Crossref] [PubMed]
- Pabinger C, Malaj I, Lothaller H, Samaila E, Magnan B. Improved Injection Technique of Ethanol for Morton’s Neuroma. Foot Ankle Int 2020;41:590-5. [Crossref] [PubMed]
- Doyle T, King K. Percutaneous removal of osteoid osteoma using CT control. Clin Radiol 1989;40:514-517. [Crossref] [PubMed]
- el-Mowafi H, Refaat H, Kotb S. Percutaneous destruction and alcoholisation for the management of osteoid osteoma. Acta Orthop Belg 2003;69:447-51.
- Adam G, Neuerburg J, Vorwerk D, Forst J, Gunther RW. Percutaneous Treatment of Osteoid Osteomas: Combonation of Drill Biopsy and Subsequent Ethanol Injection. Semin Musculoskelet Radiol 1997;1:281-4. [Crossref] [PubMed]
- Ide C, Gangi A, Rimmelin A, Beaujeux R, Maitrot D, Buchheit F, Sellal F, Dietemann JL. Vertebral haemangiomas with spinal cord compression: the place of preoperative percutaneous vertebroplasty with methyl methacrylate. Neuroradiology 1996;38:585-9. [Crossref] [PubMed]
- Gangi A, Dietemann JL, Schultz A, Mortazavi R, Jeung MY, Roy C. Interventional radiologic procedures with CT guidance in cancer pain management. Radiographics 1996;16:1289-304; discussion 1304-6. [Crossref] [PubMed]
- Gurdezi S, White T, Ramesh P. Alcohol injection for Morton’s neuroma: a five-year follow-up. Foot Ankle Int 2013;34:1064-7. [Crossref] [PubMed]
- Hughes RJ, Ali K, Jones H, Kendall S, Connell DA. Treatment of Morton’s neuroma with alcohol injection under sonographic guidance: follow-up of 101 cases. AJR Am J Roentgenol 2007;188:1535-9. [Crossref] [PubMed]
- Dockery GL. The treatment of intermetatarsal neuromas with 4% alcohol sclerosing injections. J Foot Ankle Surg 1999;38:403-8. [Crossref] [PubMed]
- Fanucci E, Masala S, Fabiano S, Perugia D, Squillaci E, Varrucciu V, Simonetti G. Treatment of intermetatarsal Morton’s neuroma with alcohol injection under US guide: 10-month follow-up. Eur Radiol 2004;14:514-8. [Crossref] [PubMed]
- Sanders P, Spouge RJ, Akbari M, Spouge DJ. Ethanol ablation of extradigital solid glomus tumors. Skeletal Radiol 2014;43:1755-9. [Crossref] [PubMed]
- Livraghi T, Giorgio A, Marin G, Salmi A, de Sio I, Bolondi L, Pompili M, Brunello F, Lazzaroni S, Torzilli G. Hepatocellular carcinoma and cirrhosis in 746 patients: long-term results of percutaneous ethanol injection. Radiology 1995;197:101-8. [Crossref] [PubMed]
- Dawson P, Becker A, Holton JM. The effect of contrast media on the growth of bacteria. Br J Radiol 1983;56:809-15. [Crossref] [PubMed]
- Bansal A, Goyal A, Gamanagatti S, Srivastava DN, Manhas V. Current updates in image-guided musculoskeletal interventions. J Clin Orthop Trauma 2021;22:101601. [Crossref] [PubMed]
- Sgalambro F, Zugaro L, Bruno F, Palumbo P, Salducca N, Zoccali C, Barile A, Masciocchi C, Arrigoni F. Interventional Radiology in the Management of Metastases and Bone Tumors. J Clin Med 2022;11:3265. [Crossref] [PubMed]
- Santiago FR, Del Mar Castellano García M, Montes JL, García MR, Fernández JM. Treatment of bone tumours by radiofrequency thermal ablation. Curr Rev Musculoskelet Med 2009;2:43-50. [Crossref] [PubMed]
- Hong K, Georgiades C. Radiofrequency ablation: mechanism of action and devices. J Vasc Interv Radiol 2010;21:S179-86. [Crossref] [PubMed]
- McGahan JP, Browning PD, Brock JM, Tesluk H. Hepatic ablation using radiofrequency electrocautery. Invest Radiol 1990;25:267-70. [Crossref] [PubMed]
- Rossi S, Fornari F, Pathies C, Buscarini L. Thermal lesions induced by 480 KHz localized current field in guinea pig and pig liver. Tumori 1990;76:54-7. [Crossref] [PubMed]
- Organ LW. Electrophysiologic principles of radiofrequency lesion making. Appl Neurophysiol 1976-1977;39:69-76. [Crossref] [PubMed]
- Goldberg SN, Hahn PF, Tanabe KK, Mueller PR, Schima W, Athanasoulis CA, Compton CC, Solbiati L, Gazelle GS. Percutaneous radiofrequency tissue ablation: does perfusion-mediated tissue cooling limit coagulation necrosis? J Vasc Interv Radiol 1998;9:101-11. [Crossref] [PubMed]
- Goldberg SN, Gazelle GS, Mueller PR. Thermal ablation therapy for focal malignancy: a unified approach to underlying principles, techniques, and diagnostic imaging guidance. AJR Am J Roentgenol 2000;174:323-31. [Crossref] [PubMed]
- Motamedi K, Levine BD, Bukata SV, Genshaft S. Percutaneous Image-Guided Musculoskeletal Tumor Treatments. AJR Am J Roentgenol 2016;207:517-25. [Crossref] [PubMed]
- Stanborough RO, Long JR, Garner HW. Bone and Soft Tissue Tumors: Interventional Techniques for Diagnosis and Treatment. Radiol Clin North Am 2022;60:311-26. [Crossref] [PubMed]
- Goldberg SN, Gazelle GS, Solbiati L, Rittman WJ, Mueller PR. Radiofrequency tissue ablation: increased lesion diameter with a perfusion electrode. Acad Radiol 1996;3:636-44. [Crossref] [PubMed]
- Livraghi T, Goldberg SN, Monti F, Bizzini A, Lazzaroni S, Meloni F, Pellicanò S, Solbiati L, Gazelle GS. Saline-enhanced radio-frequency tissue ablation in the treatment of liver metastases. Radiology 1997;202:205-10. [Crossref] [PubMed]
- Buy X, Tok CH, Szwarc D, Bierry G, Gangi A. Thermal protection during percutaneous thermal ablation procedures: interest of carbon dioxide dissection and temperature monitoring. Cardiovasc Intervent Radiol 2009;32:529-34. [Crossref] [PubMed]
- Meijerink MR, van den Tol P, van Tilborg AA, van Waesberghe JH, Meijer S, van Kuijk C. Radiofrequency ablation of large size liver tumours using novel plan-parallel expandable bipolar electrodes: initial clinical experience. Eur J Radiol 2011;77:167-71. [Crossref] [PubMed]
- Greenspan A. Benign bone-forming lesions: osteoma, osteoid osteoma, and osteoblastoma. Clinical, imaging, pathologic, and differential considerations. Skeletal Radiol 1993;22:485-500. [Crossref] [PubMed]
- Simm RJ. The natural history of osteoid osteoma. Aust N Z J Surg 1975;45:412-5. [Crossref] [PubMed]
- Kneisl JS, Simon MA. Medical management compared with operative treatment for osteoid-osteoma. J Bone Joint Surg Am 1992;74:179-85.
- Rosenthal DI, Alexander A, Rosenberg AE, Springfield D. Ablation of osteoid osteomas with a percutaneously placed electrode: a new procedure. Radiology 1992;183:29-33. [Crossref] [PubMed]
- Rosenthal DI, Hornicek FJ, Wolfe MW, Jennings LC, Gebhardt MC, Mankin HJ. Percutaneous radiofrequency coagulation of osteoid osteoma compared with operative treatment. J Bone Joint Surg Am 1998;80:815-21. [Crossref] [PubMed]
- Rimondi E, Mavrogenis AF, Rossi G, Ciminari R, Malaguti C, Tranfaglia C, Vanel D, Ruggieri P. Radiofrequency ablation for non-spinal osteoid osteomas in 557 patients. Eur Radiol 2012;22:181-8. [Crossref] [PubMed]
- Rosenthal DI, Hornicek FJ, Torriani M, Gebhardt MC, Mankin HJ. Osteoid osteoma: percutaneous treatment with radiofrequency energy. Radiology 2003;229:171-5. [Crossref] [PubMed]
- Al-Omari MH, Ata KJ, Al-Muqbel KM, Mohaidat ZM, Haddad WH, Rousan LA. Radiofrequency ablation of osteoid osteoma using tissue impedance as a parameter of osteonecrosis. J Med Imaging Radiat Oncol 2012;56:384-9. [Crossref] [PubMed]
- Akhlaghpoor S, Aziz Ahari A, Arjmand Shabestari A, Alinaghizadeh MR. Radiofrequency ablation of osteoid osteoma in atypical locations: a case series. Clin Orthop Relat Res 2010;468:1963-70. [Crossref] [PubMed]
- Cioni R, Armillotta N, Bargellini I, Zampa V, Cappelli C, Vagli P, Boni G, Marchetti S, Consoli V, Bartolozzi C. CT-guided radiofrequency ablation of osteoid osteoma: long-term results. Eur Radiol 2004;14:1203-8. [Crossref] [PubMed]
- Shields DW, Sohrabi S, Crane EO, Nicholas C, Mahendra A. Radiofrequency ablation for osteoid osteoma - Recurrence rates and predictive factors. Surgeon 2018;16:156-62. [Crossref] [PubMed]
- Martel J, Bueno A, Domínguez MP, Llorens P, Quirós J, Delgado C. Percutaneous radiofrequency ablation: relationship between different probe types and procedure time on length and extent of osteonecrosis in dog long bones. Skeletal Radiol 2008;37:147-52. [Crossref] [PubMed]
- Miyazaki M, Arai Y, Myoui A, Gobara H, Sone M, Rosenthal DI, Tsushima Y, Kanazawa S, Ehara S, Endo K. Phase I/II Multi-Institutional Study of Percutaneous Radiofrequency Ablation for Painful Osteoid Osteoma (JIVROSG-0704). Cardiovasc Intervent Radiol 2016;39:1464-70. [Crossref] [PubMed]
- Cribb GL, Goude WH, Cool P, Tins B, Cassar-Pullicino VN, Mangham DC. Percutaneous radiofrequency thermocoagulation of osteoid osteomas: factors affecting therapeutic outcome. Skeletal Radiol 2005;34:702-6. [Crossref] [PubMed]
- Ockendon M, Gregory JJ, Cribb GL, Cool WP, Mangham DC, Lalam R. Osteoid osteoma: can impedance levels in radiofrequency thermocoagulation predict recurrence? Radiol Res Pract 2011;2011:753502. [Crossref] [PubMed]
- Jaffe HL, Lichtenstein L. Benign Chondroblastoma of Bone: A Reinterpretation of the So-Called Calcifying or Chondromatous Giant Cell Tumor. Am J Pathol 1942;18:969-91.
- Robbin MR, Murphey MD. Benign chondroid neoplasms of bone. Semin Musculoskelet Radiol 2000;4:45-58. [Crossref] [PubMed]
- Atalar H, Basarir K, Yildiz Y, Erekul S, Saglik Y. Management of chondroblastoma: retrospective review of 28 patients. J Orthop Sci 2007;12:334-40. [Crossref] [PubMed]
- Rybak LD, Rosenthal DI, Wittig JC. Chondroblastoma: radiofrequency ablation--alternative to surgical resection in selected cases. Radiology 2009;251:599-604. [Crossref] [PubMed]
- Xie C, Jeys L, James SL. Radiofrequency ablation of chondroblastoma: long-term clinical and imaging outcomes. Eur Radiol 2015;25:1127-34. [Crossref] [PubMed]
- Lin PP, Thenappan A, Deavers MT, Lewis VO, Yasko AW. Treatment and prognosis of chondroblastoma. Clin Orthop Relat Res 2005;103-9. [Crossref] [PubMed]
- Lalam RK, Cribb GL, Tins BJ, Cool WP, Singh J, Tyrrell PN, Cassar-Pullicino VN. Image guided radiofrequency thermo-ablation therapy of chondroblastomas: should it replace surgery? Skeletal Radiol 2014;43:513-22. [Crossref] [PubMed]
- Okuno S. The enigma of desmoid tumors. Curr Treat Options Oncol 2006;7:438-43. [Crossref] [PubMed]
- Hosalkar HS, Torbert JT, Fox EJ, Delaney TF, Aboulafia AJ, Lackman RD. Musculoskeletal desmoid tumors. J Am Acad Orthop Surg 2008;16:188-98. [Crossref] [PubMed]
- Janinis J, Patriki M, Vini L, Aravantinos G, Whelan JS. The pharmacological treatment of aggressive fibromatosis: a systematic review. Ann Oncol 2003;14:181-90. [Crossref] [PubMed]
- Tsz-Kan T, Man-Kwong C, Shu Shang-Jen J, Ying-Lee L, Wai Man-Wah A, Hon-Shing F. Radiofrequency ablation of recurrent fibromatosis. J Vasc Interv Radiol 2007;18:147-50. [Crossref] [PubMed]
- Ilaslan H, Schils J, Joyce M, Marks K, Sundaram M. Radiofrequency ablation: another treatment option for local control of desmoid tumors. Skeletal Radiol 2010;39:169-73. [Crossref] [PubMed]
- Cobianchi L, Ravetta V, Viera FT, Filisetti C, Siri B, Segalini E, Maestri M, Dominioni T, Alessiani M, Rossi S, Dionigi P. The challenge of extraabdominal desmoid tumour management in patients with Gardner’s syndrome: radiofrequency ablation, a promising option. World J Surg Oncol 2014;12:361. [Crossref] [PubMed]
- Lutz S, Berk L, Chang E, Chow E, Hahn C, Hoskin P, Howell D, Konski A, Kachnic L, Lo S, Sahgal A, Silverman L, von Gunten C, Mendel E, Vassil A, Bruner DW, Hartsell W. Palliative radiotherapy for bone metastases: an ASTRO evidence-based guideline. Int J Radiat Oncol Biol Phys 2011;79:965-76. [Crossref] [PubMed]
- Callstrom MR, Charboneau JW, Goetz MP, Rubin J, Wong GY, Sloan JA, Novotny PJ, Lewis BD, Welch TJ, Farrell MA, Maus TP, Lee RA, Reading CC, Petersen IA, Pickett DD. Painful metastases involving bone: feasibility of percutaneous CT- and US-guided radio-frequency ablation. Radiology 2002;224:87-97. [Crossref] [PubMed]
- Goetz MP, Callstrom MR, Charboneau JW, Farrell MA, Maus TP, Welch TJ, Wong GY, Sloan JA, Novotny PJ, Petersen IA, Beres RA, Regge D, Capanna R, Saker MB, Grönemeyer DH, Gevargez A, Ahrar K, Choti MA, de Baere TJ, Rubin J. Percutaneous image-guided radiofrequency ablation of painful metastases involving bone: a multicenter study. J Clin Oncol 2004;22:300-6. [Crossref] [PubMed]
- Dupuy DE, Liu D, Hartfeil D, Hanna L, Blume JD, Ahrar K, Lopez R, Safran H, DiPetrillo T. Percutaneous radiofrequency ablation of painful osseous metastases: a multicenter American College of Radiology Imaging Network trial. Cancer 2010;116:989-97. [Crossref] [PubMed]
- Wallace AN, Greenwood TJ, Jennings JW. Radiofrequency ablation and vertebral augmentation for palliation of painful spinal metastases. J Neurooncol 2015;124:111-8. [Crossref] [PubMed]
- Gennaro N, Sconfienza LM, Ambrogi F, Boveri S, Lanza E. Thermal ablation to relieve pain from metastatic bone disease: a systematic review. Skeletal Radiol 2019;48:1161-9. [Crossref] [PubMed]
- Di Staso M, Zugaro L, Gravina GL, Bonfili P, Marampon F, Di Nicola L, Conchiglia A, Ventura L, Franzese P, Gallucci M, Masciocchi C, Tombolini V. A feasibility study of percutaneous Radiofrequency Ablation followed by Radiotherapy in the management of painful osteolytic bone metastases. Eur Radiol 2011;21:2004-10. [Crossref] [PubMed]
- Wallace AN, Robinson CG, Meyer J, Tran ND, Gangi A, Callstrom MR, Chao ST, Van Tine BA, Morris JM, Bruel BM, Long J, Timmerman RD, Buchowski JM, Jennings JW. The Metastatic Spine Disease Multidisciplinary Working Group Algorithms. Oncologist 2015;20:1205-15. [Crossref] [PubMed]
- Salama JK, Hasselle MD, Chmura SJ, Malik R, Mehta N, Yenice KM, Villaflor VM, Stadler WM, Hoffman PC, Cohen EE, Connell PP, Haraf DJ, Vokes EE, Hellman S, Weichselbaum RR. Stereotactic body radiotherapy for multisite extracranial oligometastases: final report of a dose escalation trial in patients with 1 to 5 sites of metastatic disease. Cancer 2012;118:2962-70. [Crossref] [PubMed]
- Deschamps F, Farouil G, Ternes N, Gaudin A, Hakime A, Tselikas L, Teriitehau C, Baudin E, Auperin A, de Baere T. Thermal ablation techniques: a curative treatment of bone metastases in selected patients? Eur Radiol 2014;24:1971-80. [Crossref] [PubMed]
- Welch BT, Callstrom MR, Carpenter PC, Wass CT, Welch TL, Boorjian SA, Nichols DA, Thompson GB, Lohse CM, Erickson D, Leibovich BC, Atwell TD. A single-institution experience in image-guided thermal ablation of adrenal gland metastases. J Vasc Interv Radiol 2014;25:593-8. [Crossref] [PubMed]
- Wallace AN, Tomasian A, Vaswani D, Vyhmeister R, Chang RO, Jennings JW. Radiographic Local Control of Spinal Metastases with Percutaneous Radiofrequency Ablation and Vertebral Augmentation. AJNR Am J Neuroradiol 2016;37:759-65. [Crossref] [PubMed]
- Lee FY, Latich I, Toombs C, Mungur A, Conway D, Alder K, Ibe I, Lindskog D, Friedlaender G. Minimally Invasive Image-Guided Ablation, Osteoplasty, Reinforcement, and Internal Fixation (AORIF) for Osteolytic Lesions in the Pelvis and Periarticular Regions of Weight-Bearing Bones. J Vasc Interv Radiol 2020;31:649-658.e1. [Crossref] [PubMed]
- Pinto CH, Taminiau AH, Vanderschueren GM, Hogendoorn PC, Bloem JL, Obermann WR. Technical considerations in CT-guided radiofrequency thermal ablation of osteoid osteoma: tricks of the trade. AJR Am J Roentgenol 2002;179:1633-42. [Crossref] [PubMed]
- Tomasian A, Jennings JW. Percutaneous minimally invasive thermal ablation for management of osseous metastases: recent advances. Int J Hyperthermia 2019;36:3-12. [Crossref] [PubMed]
- Chen-Xu S, Martel-Villagrán J, Bueno-Horcajadas Á. Percutaneous management of bone metastases: State of the art. Radiologia (Engl Ed) 2021;63:345-57. [Crossref] [PubMed]
- Cazzato RL, Arrigoni F, Boatta E, Bruno F, Chiang JB, Garnon J, et al. Percutaneous management of bone metastases: state of the art, interventional strategies and joint position statement of the Italian College of MSK Radiology (ICoMSKR) and the Italian College of Interventional Radiology (ICIR). Radiol Med 2019;124:34-49. [Crossref] [PubMed]
- Pusceddu C, Sotgia B, Fele RM, Melis L. Treatment of bone metastases with microwave thermal ablation. J Vasc Interv Radiol 2013;24:229-33. [Crossref] [PubMed]
- Callstrom MR, Dupuy DE, Solomon SB, Beres RA, Littrup PJ, Davis KW, Paz-Fumagalli R, Hoffman C, Atwell TD, Charboneau JW, Schmit GD, Goetz MP, Rubin J, Brown KJ, Novotny PJ, Sloan JA. Percutaneous image-guided cryoablation of painful metastases involving bone: multicenter trial. Cancer 2013;119:1033-41. [Crossref] [PubMed]
- Woo J. A short History of the development of Ultrasound in Obstetrics and Gynecology. 2002. Available online: http://www.ob-ultrasound.net/history1.html
- FRY FJ. ADES HW, FRY WJ. Production of reversible changes in the central nervous system by ultrasound. Science 1958;127:83-4. [Crossref] [PubMed]
- Lafon C, Melodelima D, Salomir R, Chapelon JY. Interstitial devices for minimally invasive thermal ablation by high-intensity ultrasound. Int J Hyperthermia 2007;23:153-63. [Crossref] [PubMed]
- Izadifar Z, Izadifar Z, Chapman D, Babyn P. An Introduction to High Intensity Focused Ultrasound: Systematic Review on Principles, Devices, and Clinical Applications. J Clin Med 2020;9:460. [Crossref] [PubMed]
- Zhou YF. High intensity focused ultrasound in clinical tumor ablation. World J Clin Oncol 2011;2:8-27. [Crossref] [PubMed]
- Lagneaux L, de Meulenaer EC, Delforge A, Dejeneffe M, Massy M, Moerman C, Hannecart B, Canivet Y, Lepeltier MF, Bron D. Ultrasonic low-energy treatment: a novel approach to induce apoptosis in human leukemic cells. Exp Hematol 2002;30:1293-301. [Crossref] [PubMed]
- ter Haar G, Rivens I, Chen L, Riddler S. High intensity focused ultrasound for the treatment of rat tumours. Phys Med Biol 1991;36:1495-501. [Crossref] [PubMed]
- Geiger D, Napoli A, Conchiglia A, Gregori LM, Arrigoni F, Bazzocchi A, Busacca M, Moreschini O, Mastantuono M, Albisinni U, Masciocchi C, Catalano C. MR-guided focused ultrasound (MRgFUS) ablation for the treatment of nonspinal osteoid osteoma: a prospective multicenter evaluation. J Bone Joint Surg Am 2014;96:743-51. [Crossref] [PubMed]
- Makin IR, Mast TD, Faidi W, Runk MM, Barthe PG, Slayton MH. Miniaturized ultrasound arrays for interstitial ablation and imaging. Ultrasound Med Biol 2005;31:1539-50. [Crossref] [PubMed]
- Salgaonkar VA, Diederich CJ. Catheter-based ultrasound technology for image-guided thermal therapy: current technology and applications. Int J Hyperthermia 2015;31:203-15. [Crossref] [PubMed]
- Cline HE, Hynynen K, Watkins RD, Adams WJ, Schenck JF, Ettinger RH, Freund WR, Vetro JP, Jolesz FA. Focused US system for MR imaging-guided tumor ablation. Radiology 1995;194:731-7. [Crossref] [PubMed]
- Hynynen K, Damianou C, Darkazanli A, Unger E, Schenck JF. The feasibility of using MRI to monitor and guide noninvasive ultrasound surgery. Ultrasound Med Biol 1993;19:91-2. [Crossref] [PubMed]
- Jolesz FA, McDannold N. Current status and future potential of MRI-guided focused ultrasound surgery. J Magn Reson Imaging 2008;27:391-9. [Crossref] [PubMed]
- Napoli A, Anzidei M, Marincola BC, Brachetti G, Noce V, Boni F, Bertaccini L, Passariello R, Catalano C. MR imaging-guided focused ultrasound for treatment of bone metastasis. Radiographics 2013;33:1555-68. [Crossref] [PubMed]
- Miller DL, Smith NB, Bailey MR, Czarnota GJ, Hynynen K, Makin IR. Overview of therapeutic ultrasound applications and safety considerations. J Ultrasound Med 2012;31:623-34. [Crossref] [PubMed]
- Hurwitz MD, Ghanouni P, Kanaev SV, Iozeffi D, Gianfelice D, Fennessy FM, Kuten A, Meyer JE, LeBlang SD, Roberts A, Choi J, Larner JM, Napoli A, Turkevich VG, Inbar Y, Tempany CM, Pfeffer RM. Magnetic resonance-guided focused ultrasound for patients with painful bone metastases: phase III trial results. J Natl Cancer Inst 2014;106:dju082. [Crossref] [PubMed]
- Catane R, Beck A, Inbar Y, Rabin T, Shabshin N, Hengst S, Pfeffer RM, Hanannel A, Dogadkin O, Liberman B, Kopelman D. MR-guided focused ultrasound surgery (MRgFUS) for the palliation of pain in patients with bone metastases--preliminary clinical experience. Ann Oncol 2007;18:163-7. [Crossref] [PubMed]
- Gianfelice D, Gupta C, Kucharczyk W, Bret P, Havill D, Clemons M. Palliative treatment of painful bone metastases with MR imaging--guided focused ultrasound. Radiology 2008;249:355-63. [Crossref] [PubMed]
- Liberman B, Gianfelice D, Inbar Y, Beck A, Rabin T, Shabshin N, Chander G, Hengst S, Pfeffer R, Chechick A, Hanannel A, Dogadkin O, Catane R. Pain palliation in patients with bone metastases using MR-guided focused ultrasound surgery: a multicenter study. Ann Surg Oncol 2009;16:140-6. [Crossref] [PubMed]
- Napoli A, Anzidei M, Marincola BC, Brachetti G, Ciolina F, Cartocci G, Marsecano C, Zaccagna F, Marchetti L, Cortesi E, Catalano C. Primary pain palliation and local tumor control in bone metastases treated with magnetic resonance-guided focused ultrasound. Invest Radiol 2013;48:351-8. [Crossref] [PubMed]
- Samardziski M, Zafiroski G, Tolevska C, Zafirova-Ivanovska B, Kostadinova-Kunovska S, Kalicanin-Markovska M. Limb-sparing in patients with non-metastatic high-grade osteosarcoma. J BUON 2009;14:63-9.
- Chen W, Zhu H, Zhang L, Li K, Su H, Jin C, Zhou K, Bai J, Wu F, Wang Z. Primary bone malignancy: effective treatment with high-intensity focused ultrasound ablation. Radiology 2010;255:967-78. [Crossref] [PubMed]
- Li C, Zhang W, Fan W, Huang J, Zhang F, Wu P. Noninvasive treatment of malignant bone tumors using high-intensity focused ultrasound. Cancer 2010;116:3934-42. [Crossref] [PubMed]
- Temple MJ, Waspe AC, Amaral JG, Napoli A, LeBlang S, Ghanouni P, Bucknor MD, Campbell F, Drake JM. Establishing a clinical service for the treatment of osteoid osteoma using magnetic resonance-guided focused ultrasound: overview and guidelines. J Ther Ultrasound 2016;4:16. [Crossref] [PubMed]
- Napoli A, Mastantuono M, Cavallo Marincola B, Anzidei M, Zaccagna F, Moreschini O, Passariello R, Catalano C. Osteoid osteoma: MR-guided focused ultrasound for entirely noninvasive treatment. Radiology 2013;267:514-21. [Crossref] [PubMed]
- Arrigoni F, Napoli A, Bazzocchi A, Zugaro L, Scipione R, Bruno F, Palumbo P, Anzidei M, Mercatelli D, Gravina GL, Zoccali C, Ghanouni P, Barile A, Catalano C, Masciocchi C. Magnetic-resonance-guided focused ultrasound treatment of non-spinal osteoid osteoma in children: multicentre experience. Pediatr Radiol 2019;49:1209-16. [Crossref] [PubMed]
- Wang Y, Wang W, Tang J. Ultrasound-guided high intensity focused ultrasound treatment for extra-abdominal desmoid tumours: preliminary results. Int J Hyperthermia 2011;27:648-53. [Crossref] [PubMed]
- Avedian RS, Bitton R, Gold G, Butts-Pauly K, Ghanouni P. Is MR-guided High-intensity Focused Ultrasound a Feasible Treatment Modality for Desmoid Tumors? Clin Orthop Relat Res 2016;474:697-704. [Crossref] [PubMed]
- Ghanouni P, Dobrotwir A, Bazzocchi A, Bucknor M, Bitton R, Rosenberg J, Telischak K, Busacca M, Ferrari S, Albisinni U, Walters S, Gold G, Ganjoo K, Napoli A, Pauly KB, Avedian R. Magnetic resonance-guided focused ultrasound treatment of extra-abdominal desmoid tumors: a retrospective multicenter study. Eur Radiol 2017;27:732-40. [Crossref] [PubMed]
- Yan X, Zhao C, Tian C, Wen S, He X, Zhou Y. Ultrasound-guided high-intensity focused ultrasound ablation for treating uterine arteriovenous malformation. BJOG 2017;124:93-6. [Crossref] [PubMed]
- Rieck B, Bates D, Zhang K, Escott N, Mougenot C, Pichardo S, Curiel L. Focused ultrasound treatment of abscesses induced by methicillin resistant Staphylococcus aureus: feasibility study in a mouse model. Med Phys 2014;41:063301. [Crossref] [PubMed]
- Kurup AN, Schmit GD, Morris JM, Atwell TD, Schmitz JJ, Weisbrod AJ, Woodrum DA, Eiken PW, Callstrom MR. Avoiding Complications in Bone and Soft Tissue Ablation. Cardiovasc Intervent Radiol 2017;40:166-76. [Crossref] [PubMed]
- Tsoumakidou G, Buy X, Garnon J, Enescu J, Gangi A. Percutaneous thermal ablation: how to protect the surrounding organs. Tech Vasc Interv Radiol 2011;14:170-6. [Crossref] [PubMed]
- Kurup AN, Morris JM, Boon AJ, Strommen JA, Schmit GD, Atwell TD, Carter RE, Brown MJ, Wass CT, Rose PS, Callstrom MR. Motor evoked potential monitoring during cryoablation of musculoskeletal tumors. J Vasc Interv Radiol 2014;25:1657-64. [Crossref] [PubMed]
- Tsoumakidou G, Garnon J, Ramamurthy N, Buy X, Gangi A. Interest of electrostimulation of peripheral motor nerves during percutaneous thermal ablation. Cardiovasc Intervent Radiol 2013;36:1624-8. [Crossref] [PubMed]
- Chiras J, Clarencon F, Cormier E, Jean L. Radiologie interventionnelle des metástases osseuses. Oncologie 2015;107-16.
- Galibert P, Deramond H, Rosat P, Le Gars D. Preliminary note on the treatment of vertebral angioma by percutaneous acrylic vertebroplasty. Neurochirurgie 1987;33:166-8.
- Weill A, Chiras J, Simon JM, Rose M, Sola-Martinez T, Enkaoua E. Spinal metastases: indications for and results of percutaneous injection of acrylic surgical cement. Radiology 1996;199:241-7. [Crossref] [PubMed]
- Dean JR, Ison KT, Gishen P. The strengthening effect of percutaneous vertebroplasty. Clin Radiol 2000;55:471-6. [Crossref] [PubMed]
- Nelson DA, Barker ME, Hamlin BH. Thermal effects of acrylic cementation at bone tumour sites. Int J Hyperthermia 1997;13:287-306. [Crossref] [PubMed]
- Buy X, Catena V, Roubaud G, Crombe A, Kind M, Palussiere J. Image-Guided Bone Consolidation in Oncology. Semin Intervent Radiol 2018;35:221-8. [Crossref] [PubMed]
- Baroud G, Samara M, Steffen T. Influence of mixing method on the cement temperature-mixing time history and doughing time of three acrylic cements for vertebroplasty. J Biomed Mater Res B Appl Biomater 2004;68:112-6. [Crossref] [PubMed]
- Belkoff SM, Molloy S. Temperature measurement during polymerization of polymethylmethacrylate cement used for vertebroplasty. Spine (Phila Pa 1976) 2003;28:1555-9.
- Sutter EG, Mears SC, Belkoff SM. A biomechanical evaluation of femoroplasty under simulated fall conditions. J Orthop Trauma 2010;24:95-9. [Crossref] [PubMed]
- Weill A, Kobaiter H, Chiras J. Acetabulum malignancies: technique and impact on pain of percutaneous injection of acrylic surgical cement. Eur Radiol 1998;8:123-9. [Crossref] [PubMed]
- Gupta AC, Hirsch JA, Chaudhry ZA, Chandra RV, Pulli B, Galinsky JG, Hirsch AE, Yoo AJ. Evaluating the safety and effectiveness of percutaneous acetabuloplasty. J Neurointerv Surg 2012;4:134-8. [Crossref] [PubMed]
- Heini PF, Franz T, Fankhauser C, Gasser B, Ganz R. Femoroplasty-augmentation of mechanical properties in the osteoporotic proximal femur: a biomechanical investigation of PMMA reinforcement in cadaver bones. Clin Biomech (Bristol, Avon) 2004;19:506-12. [Crossref] [PubMed]
- Katsanos K, Sabharwal T, Adam A. Percutaneous cementoplasty. Semin Intervent Radiol 2010;27:137-47. [Crossref] [PubMed]
- Tsoumakidou G, Too CW, Koch G, Caudrelier J, Cazzato RL, Garnon J, Gangi A. CIRSE Guidelines on Percutaneous Vertebral Augmentation. Cardiovasc Intervent Radiol 2017;40:331-42. [Crossref] [PubMed]
- Caudana R, Renzi Brivio L, Ventura L, Aitini E, Rozzanigo U, Barai G. CT-guided percutaneous vertebroplasty: personal experience in the treatment of osteoporotic fractures and dorsolumbar metastases. Radiol Med 2008;113:114-33. [Crossref] [PubMed]
- Vogl TJ, Proschek D, Schwarz W, Mack M, Hochmuth K. CT-guided percutaneous vertebroplasty in the therapy of vertebral compression fractures. Eur Radiol 2006;16:797-803. [Crossref] [PubMed]
- Moser TP, Onate M, Achour K, Freire V. Cementoplasty of pelvic bone metastases: systematic assessment of lesion filling and other factors that could affect the clinical outcomes. Skeletal Radiol 2019;48:1345-55. [Crossref] [PubMed]
- Garnon J, Meylheuc L, Cazzato RL, Dalili D, Koch G, Auloge P, Bayle B, Gangi A. Percutaneous extra-spinal cementoplasty in patients with cancer: A systematic review of procedural details and clinical outcomes. Diagn Interv Imaging 2019;100:743-52. [Crossref] [PubMed]
- Kurup AN, Morris JM, Schmit GD, Atwell TD, Schmitz JJ, Rose PS, Callstrom MR. Balloon-assisted osteoplasty of periacetabular tumors following percutaneous cryoablation. J Vasc Interv Radiol 2015;26:588-94. [Crossref] [PubMed]
- Cazzato RL, Garnon J, Caudrelier J, Rao PP, Koch G, Gangi A. Percutaneous radiofrequency ablation of painful spinal metastasis: a systematic literature assessment of analgesia and safety. Int J Hyperthermia 2018;34:1272-81. [Crossref] [PubMed]
- Kam NM, Maingard J, Kok HK, Ranatunga D, Brooks D, Torreggiani WC, Munk PL, Lee MJ, Chandra RV, Asadi H. Combined Vertebral Augmentation and Radiofrequency Ablation in the Management of Spinal Metastases: an Update. Curr Treat Options Oncol 2017;18:74. [Crossref] [PubMed]
- Hoffmann RT, Jakobs TF, Trumm C, Weber C, Helmberger TK, Reiser MF. Radiofrequency ablation in combination with osteoplasty in the treatment of painful metastatic bone disease. J Vasc Interv Radiol 2008;19:419-25. [Crossref] [PubMed]
- Munk PL, Rashid F, Heran MK, Papirny M, Liu DM, Malfair D, Badii M, Clarkson PW. Combined cementoplasty and radiofrequency ablation in the treatment of painful neoplastic lesions of bone. J Vasc Interv Radiol 2009;20:903-11. [Crossref] [PubMed]
- Lane MD, Le HB, Lee S, Young C, Heran MK, Badii M, Clarkson PW, Munk PL. Combination radiofrequency ablation and cementoplasty for palliative treatment of painful neoplastic bone metastasis: experience with 53 treated lesions in 36 patients. Skeletal Radiol 2011;40:25-32. [Crossref] [PubMed]
- Sun G, Jin P, Liu XW, Li M, Li L. Cementoplasty for managing painful bone metastases outside the spine. Eur Radiol 2014;24:731-7. [Crossref] [PubMed]
- Cornelis FH, Deschamps F. Augmented osteoplasty for proximal femur consolidation in cancer patients: Biomechanical considerations and techniques. Diagn Interv Imaging 2017;98:645-50. [Crossref] [PubMed]
- Cazzato RL, Koch G, Garnon J, Ramamurthy N, Jégu J, Clavert P, Gangi A. Biomechanical effects of osteoplasty with or without Kirschner wire augmentation on long bone diaphyses undergoing bending stress: implications for percutaneous imaging-guided consolidation in cancer patients. Eur Radiol Exp 2019;3:4. [Crossref] [PubMed]
- Deschamps F, Farouil G, Hakime A, Barah A, Guiu B, Teriitehau C, Auperin A, deBaere T. Cementoplasty of metastases of the proximal femur: is it a safe palliative option? J Vasc Interv Radiol 2012;23:1311-6. [Crossref] [PubMed]
- Filippiadis DK, Tselikas L, Bazzocchi A, Efthymiou E, Kelekis A, Yevich S. Percutaneous Management of Cancer Pain. Curr Oncol Rep 2020;22:43. [Crossref] [PubMed]
- Cortet B, Cotten A, Deprez X, Deramond H, Lejeune JP, Leclerc X, Chastanet P, Duquesnoy B, Delcambre B. Value of vertebroplasty combined with surgical decompression in the treatment of aggressive spinal angioma. Apropos of 3 cases. Rev Rhum Ed Fr 1994;61:16-22.
- Cotten A, Boutry N, Cortet B, Assaker R, Demondion X, Leblond D, Chastanet P, Duquesnoy B, Deramond H. Percutaneous vertebroplasty: state of the art. Radiographics 1998;18:311-20; discussion 320-3. [Crossref] [PubMed]
- Cotten A, Duquesnoy B. Percutaneous cementoplasty for malignant osteolysis of the acetabulum. Presse Med 1995;24:1308-10.
- Wang WG, Wu CG, Gu YF, Li MH. Percutaneous osteoplasty for the management of a femoral head metastasis: a case report. Korean J Radiol 2009;10:641-4. [Crossref] [PubMed]
- Anselmetti GC. Osteoplasty: Percutaneous Bone Cement Injection beyond the Spine. Semin Intervent Radiol 2010;27:199-208. [Crossref] [PubMed]
- Mirels H. Metastatic disease in long bones. A proposed scoring system for diagnosing impending pathologic fractures. Clin Orthop Relat Res 1989;256-64.
- Gangi A, Guth S, Imbert JP, Marin H, Dietemann JL. Percutaneous vertebroplasty: indications, technique, and results. Radiographics 2003;23:e10. [Crossref] [PubMed]
- Peh WC, Munk PL, Rashid F, Gilula LA. Percutaneous vertebral augmentation: vertebroplasty, kyphoplasty and skyphoplasty. Radiol Clin North Am 2008;46:611-35. vii. [Crossref] [PubMed]
- Nussbaum DA, Gailloud P, Murphy K. A review of complications associated with vertebroplasty and kyphoplasty as reported to the Food and Drug Administration medical device related web site. J Vasc Interv Radiol 2004;15:1185-92. [Crossref] [PubMed]
- Pérez-Serrano C, Vollmer I, Perea RJ. The Utility Of Dual-Energy Computed Tomography In The Diagnosis Of Pulmonary Thromboembolism Caused By Post-Vertebroplasty Cement: A Case Report. Arch Bronconeumol (Engl Ed) 2018;54:155. [Crossref] [PubMed]
- Scroop R, Eskridge J, Britz GW. Paradoxical cerebral arterial embolization of cement during intraoperative vertebroplasty: case report. AJNR Am J Neuroradiol 2002;23:868-70.
- Kodama H, Aikata H, Uka K, Takaki S, Mori N, Waki K, Jeong SC, Kawakami Y, Shirakawa H, Takahashi S, Toyota N, Ito K, Chayama K. Efficacy of percutaneous cementoplasty for bone metastasis from hepatocellular carcinoma. Oncology 2007;72:285-92. [Crossref] [PubMed]
- Gangi A, Sabharwal T, Irani FG, Buy X, Morales JP, Adam A. Quality assurance guidelines for percutaneous vertebroplasty. Cardiovasc Intervent Radiol 2006;29:173-8. [Crossref] [PubMed]
- Cloft HJ, Easton DN, Jensen ME, Kallmes DF, Dion JE. Exposure of medical personnel to methylmethacrylate vapor during percutaneous vertebroplasty. AJNR Am J Neuroradiol 1999;20:352-3.
- Mohme M, Riethdorf S, Dreimann M, Werner S, Maire CL, Joosse SA, Bludau F, Mueller V, Neves RPL, Stoecklein NH, Lamszus K, Westphal M, Pantel K, Wikman H, Eicker SO. Circulating Tumour Cell Release after Cement Augmentation of Vertebral Metastases. Sci Rep 2017;7:7196. [Crossref] [PubMed]
- McGraw JK, Cardella J, Barr JD, Mathis JM, Sanchez O, Schwartzberg MS, Swan TL, Sacks D. Society of Interventional Radiology quality improvement guidelines for percutaneous vertebroplasty. J Vasc Interv Radiol 2003;14:827-31. [Crossref] [PubMed]
- Cazzato RL, Garnon J, Shaygi B, Boatta E, Koch G, Palussiere J, Buy X, Gangi A. Percutaneous consolidation of bone metastases: strategies and techniques. Insights Imaging 2019;10:14. [Crossref] [PubMed]