The effect of reducing spatial resolution by in-plane partial volume averaging on peak velocity measurements in phase contrast magnetic resonance angiography
Introduction
Cardiac magnetic resonance (CMR) imaging has several attractions as an imaging modality; it is non-invasive, can be conducted without intravenous contrast medium or the use of ionizing radiation and can produce useful physiological data. Phase contrast magnetic resonance angiography (PCMRA) is a validated method for in vivo measurement of flow volume within blood vessels (1). It has a variety of established cardiac clinical applications, such as calculating cardiac output, shunt flow (2,3) and aortic or pulmonary regurgitation (4,5). Detecting the maximum velocity of flow across a stenosis is important in determining the severity of a stenotic lesion. In all these scenarios, electrocardiograph (ECG) gated PCMRA is used to produce a data set encompassing flow in the vessel of interest at multiple time points over the cardiac cycle (6). This data set is then analyzed to assess velocity and volume of flow during a single averaged heartbeat. In general, as the vessels of interest are large compared to the size of the voxels in the field of view, the volume of flow recorded is representative (6). However, determining the true peak velocity within a vessel is more difficult, as this may occur in a small region and over a short time period only, for example at the aortic valve (AorV) tip during systole. The ability to detect the highest velocity in a data set will thus be influenced by both the spatial and temporal resolution of the image. PCMRA can be used to calculate peak velocity. It is expected that higher velocity will be recorded when spatial resolution is optimized. The aim of this study was to quantify the effect of in-plane partial volume averaging on recorded peak velocity in PCMRA. The reason for this is that:
- Manufactures of software for the analysis of flow imaging (for example Argus (Siemens Medical Solutions, Erlangen, Germany) and CVI 42 (Circle Cardiovascular Imaging Inc, Calgary, Canada) offer a variety of voxel averaged and non-voxel averaged outputs for peak velocity; and the effect of this has not been previously documented;
- The literature is inconsistent about the use of voxel averaging with (7-12) not defining whether it was used, using single voxel (13,14) and using voxel averaging (15).
Methods
Study population
The peak velocity in 145 flow measurements in 14 different anatomical locations in 37 subjects who had undergone PCMRA were retrospectively identified and included in the study (Tables 1,2). Patients with acquired heart disease included subjects with cardiomyopathy, pulmonary artery hypertension, unexplained ventricular dilatation and valvular heart disease. Patients with congenital heart disease included subjects with atrial and ventricular septal defect, coarctation (and post repair), bicuspid AorV, post pulmonary valvotomy, pulmonary arterial hypoplasia; post-surgical repair of hypoplastic left heart syndrome, transposition of the great arteries, tetralogy of Fallot and double outlet right ventricle. All flows were performed in the short axis plane of the vessel of interest. This was obtained by aligning the PCMRA plane at right angles to two perpendicular long axis planes of the vessel. This study was conducted as a retrospective evaluation of service delivery and as such did not require full ethics approval following consultation with the Chairman of the National Research Ethics Service: South West—Central Bristol and in accordance with the National Health Service Research Ethics Service guidelines (16).

Full table
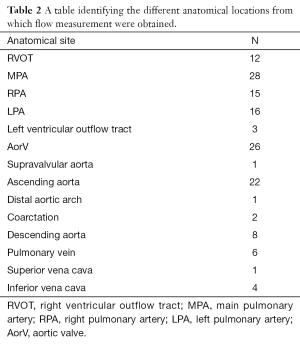
Full table
CMR systems tested and scan protocols
The study was limited to 1.5 Tesla, the most widely used main field strength for clinical CMR. The studies identified had been performed on either a Siemens Symphony or Siemens Avanto CMR scanner (Siemens Medical Solutions, Erlangen, Germany) with cardiac phased array coils. The following scan parameters were employed:
- Avanto breath held flow. Time to repeat (TR) 47.1 ms; time to echo (TE) 2.0 ms; field of view 220 mm × 320 mm (standard but altered depending on patient size); velocity encoding altered to match expected peak flow; 20 reconstructions per heartbeat. Slice thickness 5.5 mm. Voxel size 1.7 mm × 1.7 mm ×5.5 mm. Number of signals averaged =1;
- Avanto free breathing. TR 29.9 ms with TE 2.2 ms (standard) or TR 31.6 ms with TE 2.5 ms or TR 39.4 ms and TE 3.5 ms; field of view 220 mm × 320 mm (standard but altered depending on patient size); velocity encoding altered to match expected peak flow; 30 reconstructions per heartbeat. Slice thickness 5 mm. Voxel size 1.3 mm × 1.3 mm ×5 mm. Number of signals averaged =3;
- Symphony breath held flow. TR 58 ms with TE 3.0 ms (standard) or TR 46.5 ms and TE 3.0 ms or TR 37.4 ms and TE 3.1 ms or TR 23.4 ms and TE 3.0 ms; field of view 220 mm × 320 mm (standard but altered depending on patient size); velocity encoding altered to match expected peak flow; 20 reconstructions per heartbeat. Slice thickness 5.5 mm. Voxel size 1.7 mm × 1.7 mm × 5.5 mm. Number of signals averaged =1;
- Symphony free breathing flow. TR 36.9 ms; with TE 3.1 ms (standard); field of view 240 mm × 320 mm (standard but altered depending on patient size); velocity encoding altered to match expected peak flow; 30 reconstructions per heartbeat. Slice thickness 5 mm. Voxel size 1.3 mm × 1.3 mm ×5 mm. Number of signals averaged =3.
All flow measurements were performed perpendicular to the vessel or valve of interest.
CMR analysis
Flow analysis was performed by experienced CMR readers using Argus flow quantification software (Siemens Medical Solutions, Erlangen, Germany). A region of interest (ROI) was manually drawn to encompass all the flow in the vessel of interest. The ROI was carefully adjusted to exclude noise from outside the vessel. This ROI was then propagated and the contours manually adjusted through all the images encompassing the cardiac cycle. When calculating the peak velocity, the standard Argus software displays the data as an average of the peak velocity voxel and its neighbouring four voxels. Specifically, for every voxel in the ROI, the software calculates a new value which is the average of the nominal voxel and the voxels immediately adjacent anterior, posterior, left and right as long as these voxels are within the drawn ROI. This is done for every voxel in the ROI and the peak velocity is re-calculated from these average values. The voxel averaged peak velocity and overall flow was recorded. Then, the voxel averaging function was disabled, via the flow options tab, and the data was re-recorded. Care was taken not to record noise as the peak velocity by checking that the new peak velocity was recorded from within the appropriate region, both anatomically and by temporal window.
Statistical analysis
Statistical analysis was performed using SPSS v.21 (Armonk, NY, USA: IBM Corp). The presence of a normal distribution was tested with the Shapiro-Wilk test. Normally distributed paired data were compared with the two-tailed paired student T test and non-normally distributed paired data were compared with the Wilcoxon paired signed rank test. The Kruskall-Wallis test was used to assess for significant differences between voxel-averaging and single-voxel techniques at different anatomical locations and to assess for significant differences in mean percentage difference between peak velocity subgroups. Significance was set at two-tailed P<0.05.
Results
Demographics
One hundred and fourty-five flow measurements were obtained from a total of 37 individuals and from 14 different anatomical sites (Tables 1,2).
Effect of voxel averaging on peak velocity measurement
The peak velocity measurements calculated using the single peak velocity voxel method were higher than when the averaged method was employed (Figure 1). The difference reached high statistical significance (Figure 2). The mean absolute difference between peak velocities calculated using the two methods was 8.5 cm.s−1. This equates to a mean percentage increase in peak velocity of 7.1%, when the single voxel technique was used as opposed to the voxel averaging technique.
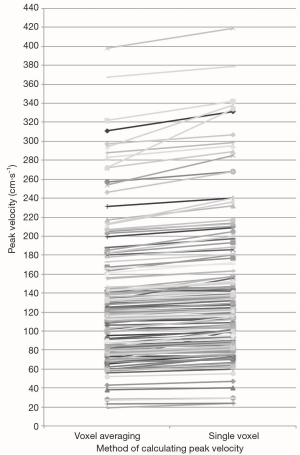
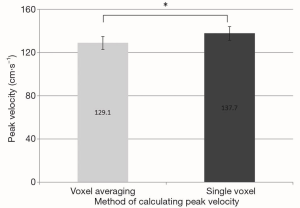
The peak velocity measurements were significantly higher when calculated using the single voxel method in comparison to the averaged method at all anatomical sites within the pulmonary vasculature (Figure 3) and within the thoracic aorta (Figure 4).
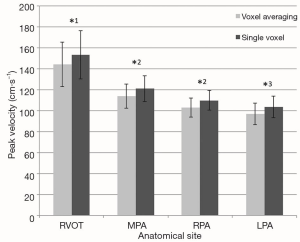
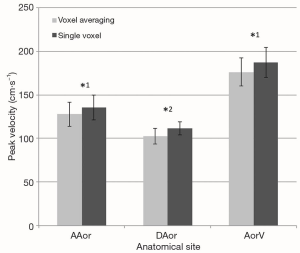
Effect of voxel averaging on peak velocity by MR scanner type
The mean percentage change in peak velocity calculated by the averaged and single voxel methods on the Avanto MR scanner was 6.4%±0.4% (n=52) compared to 8.4%±1.2% on scans performed on the Symphony MR machine (n=93). This difference was not statistically significant (P=0.069).
Effect of voxel averaging on peak velocity by breathing instruction
The mean percentage change in peak velocity calculated by the averaged and single voxel methods for scans performed with free breathing was 6.7%±0.8% (n=52) compared to 7.3%±0.6% (n=93) on scans performed under breath hold. This difference did not reach statistical significance (P=0.510).
Effect of voxel averaging on peak velocity by velocity subgroup
There is a mean increase in the calculated peak velocity of 5–10% in all peak velocity subgroups (Tables 3,4).

Full table
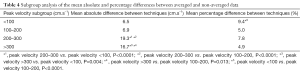
Full table
Discussion
Our study demonstrated that there was a highly significant increase in calculated peak velocity when a single voxel measurement technique was employed in comparison to the voxel averaging method. As this is the result of how the data is displayed it was not surprising that this was regardless of the type of patient scanned, anatomical location, MRI scanner type or scan technique in terms of breathing command. Disabling the voxel averaging technique had no effect on the volume of flow recorded. This paper was not designed to assess the effect of TE, slice position and turbulence on the assessment of velocity, variables which have previously been assessed (13,14). Nor was it designed to directly compare the technique to transthoracic echocardiography (TTE). While it is not surprising that the single voxel technique produced a higher recorded peak velocity (by improving the effective spatial resolution without averaging with adjacent voxels with lower recorded velocities) it is important to know the scale of the improvement in peak velocity measurement. This is underlined by the fact that many papers on the velocity assessment of AorV stenosis by PCMRA do not mention whether or not voxel averaging has been employed and different software vendors allow different degrees of voxel averaging. This suggests that much of the previous literature that addresses peak velocity estimation in MRI has neglected an important and basic factor that influences the results.
Indeed we observed a 7% mean percentage increase in peak velocity when the single voxel technique was employed compared to the voxel averaging technique. This is unhelpful if PCMRA is being used to assess the peak velocity and estimate the peak pressure drop (PPD) between two chambers. The PPD can be estimated by the simplified Bernoulli equation (PPD =4V2, where V = peak velocity). When the peak velocity calculated with voxel averaged PCMRA is small, e.g., V=1 m.s−1, with a 7% velocity underestimation, the true peak will actually be 1.07 m.s−1. The true PDD will be therefore be 4.6 mmHg (i.e., 4×1.072) compared to the PPD calculated with the voxel averaged PCMRA peak velocity of 4 mmHg (i.e., 4×1.02). This difference of 0.6 mmHg is of no clinical significance. However, at higher peak velocities, a small unnecessary underestimation in peak velocity may become clinically relevant. For example, if the recorded velocity is 3.9 m.s−1, the PPD will be 60.8 mmHg (i.e., 4×3.92). Taking into account the 7% underestimation, the likely true peak recorded velocity would have been 4.17 m.s−1 and the true PPD will be 69.6 mmHg (i.e., 4×4.172). This could lead to severe AorV stenosis (V>4 m.s−1) being misclassified as moderate (V<4 m.s−1).
When measuring peak velocity the observer should be aware whether or not voxel averaging is used, as this will reduce the effective spatial resolution of the measurement and reduce the recorded peak velocity. For example Argus flow analysis defaults to averaging the peak recorded velocity with the surrounding four voxels. It is not clear whether researchers who use this widely distributed platform are aware of this default. Recent reviews of the use of PCMRA for the assessment of valvular disease described the importance of minimizing slice thickness to reduce the effect of partial volume averaging but do not mention the importance of optimization of in-plane voxel size by ensuring single voxel methods are employed (11,17). While the voxel averaging technique produces a less noisy pictorial display of flow over the cardiac cycle, it systematically leads to underestimation of the measured peak velocity. Although only two CMR machines from one vendor were assessed in this study, the effect of partial volume averaging in calculating accurate peak velocities is determined by the parameters, which define the size of the voxel, which are not vendor specific. As such, the same issues are likely to apply to different manufacturers of different CMR machines, if their post-processing software calculates peak velocity in the same manner.
While PCMRA is robust and widely used in clinical practice for assessing volume of flow, it has been less useful at determining the peak velocity of flow. This is in part due to a combination of lower temporal and spatial resolution when compared with continuous wave Doppler ultrasound, an established technique for measuring peak velocity with both high spatial and temporal resolution (18). Comparing PCMRA with Doppler ultrasound in the assessment of peak velocity has been documented without voxel averaging (9,19) where good correlation is claimed, and with voxel averaging (15), where a systematic underestimation of peak velocity was noted with PCMRA. The aim of this study was to quantify the precise scale of the effect of voxel averaging and thus explain why an improvement in in-plane volume average by using a single voxel technique to calculate peak velocity might improve the accuracy of PCMRA relative to TTE Doppler.
There are several potential technical and patient sources of error, which may lead to underestimation of the estimated peak velocity in PCMRA, such as the effects of acceleration in jet, flow turbulence and incorrect slice angulation that are not perpendicular to the flow jet. In our analysis, all flow measurements were performed perpendicular to the vessel or valve of interest, which will have led to underestimation of very eccentric jets. Reducing the effect of partial volume averaging can be achieved by minimizing the field of view to maximize spatial resolution. By additionally employing a single rather than multiple voxel technique to calculate peak velocity we will also improve the measurement of peak velocity by improving the spatial resolution of the displayed velocity dataset.
A potential problem with PCMRA is the effect of noise within the dataset. Noise is related to the scale of the velocity encoding (with higher encoding introducing more noise) and the signal to noise ratio (SNR) (6). Noise was minimized by the velocity encoding being well matched to the expected peak velocity (typically 1–1.5 m/s, increased if there was aliasing). Of interest the mean underestimation of velocity did not systematically increase with increasing velocity encoding, suggesting this effect was not significant. Regarding signal to noise, minimizing the effect of volume averaging must be weighed against any potential adverse effects of reducing the SNR but this is difficult to quantify, particularly in pulsatile in vivo flow. As there is no standard method of measuring SNR in flow imaging and thus no recognized ‘good’ range of value for SNR we were unable to conduct a meaningful analysis though it is noteworthy that these sequences produce high signal (with low noise) in systole.
Significant outliers are removed by ensuring that the recorded peak velocity occurs within high quality signal, away from the edge voxel and at peak systole (by timing and flow rate). Random fluctuations in noise related to the voxel will only systematically cause an increase in peak velocity estimation across the whole of this study if the velocity of flow is truly laminar with the same velocity across the whole vessel. This is not the case in vessels. In vessels there is a range of flow rates across the vessel. This means that noise in the voxel with the highest velocity is as likely to have a positive as a negative effect.
The derived peak velocity curves can be viewed to determine if they are smooth (as per an optimal continuous wave Doppler trace) or jagged and potentially invalid. Without a standard for assessing noise however the true effect cannot be determined. However this problem is also shared by Doppler, where in addition to (also unquantified) noise effects the peak velocity is not derived by the flow curve it, but by the observer’s visual interpretation of where to mark the peak velocity, which itself could lead to substantial bias. Finally there is a further bias towards higher velocity estimation with Doppler. This is that PCMRA peak velocity is estimated over many heartbeats, whereas with Doppler it is common practice to only measure the heartbeat with the highest velocity and certainly rare to average over more than a few heartbeats.
The reliability of PCMRA has previously been validated (1). The flow analysis was performed by four experienced CMR readers. Inter and intra observer variability was not calculated in this retrospective study. Furthermore, slight potential variability in data between subjects due to human error in analysis is not of particular relevance in the current study, which was designed to assess for change in peak velocity within an individual subject’s dataset when analyzed with two different techniques. In this study, each patient essentially acted as their own control. Moreover, this was a proof of concept study and not a validation study of a new analytical technique. Ultimately, there is no scope for variation associated with human error between the two peak velocity measurements recorded per subject because the ROI did not change between recording the data in default voxel averaging setting and then re-recording the data using the single voxel technique.
All techniques that quantify blood flow velocity in vivo are hindered by the lack of a standard of reference. In PCMRA, flow measurements are only approximations based on results of phantom studies. In theory, using the Gorlin formula with invasive catheterisation data could have been used as a comparator. However, such an invasive examination is unlikely to have been granted ethics approval, especially in our target subject population with aortic stenosis, who are at increased risk of cerebrovascular complications of such procedures (20). This study was not designed to compare PCMRA with Doppler ultrasound. Rather, it was designed to determine whether we are under-estimating the recorded peak velocity by using voxel averaging rather than a single voxel peak velocity technique. Although we have alluded to the clinical relevance of our findings, the absence of an independent gold standard peak velocity measurement render it difficult to assess if the volume averaging effects we have demonstrated contribute in a major or minor way to the bias of PCMRA and further research is required to address this question.
Conclusions
Reducing spatial resolution by the use of voxel averaging produces a consistent underestimation of peak velocity in PCMRA as compared to the single voxel technique. If it is understood that reducing spatial resolution is likely to lead to underestimation of velocity this in itself is not surprising. Previous studies have neglected this important variable and this is the first study to assess the amount the recorded velocity changes and provides important data to those analysing PCMRA who may not be aware of the effect of pixel averaging on their results. As well as optimizing factors such as field of view, slice thickness, position and TE, the use of voxel averaging should be avoided as the observer should, when estimating peak velocity, be optimizing both the spatial resolution of the image as well as the spatial resolution of the recorded velocity dataset. Further research needs to be conducted to assess the clinical implication, although our results suggest that this phenomenon may, at least in part, explain the trend towards lower peak velocities with PCMRA in comparison to Doppler echocardiography.
Acknowledgements
None.
Footnote
Conflicts of Interest: The authors have no conflicts of interest to declare.
Ethical Statement: The study was approved by the South West—Central Bristol Research Ethics Committee. As a retrospective review of service delivery no informed consent was required.
References
- Powell AJ, Maier SE, Chung T, Geva T. Phase-velocity cine magnetic resonance imaging measurement of pulsatile blood flow in children and young adults: in vitro and in vivo validation. Pediatr Cardiol 2000;21:104-10. [Crossref] [PubMed]
- Petersen SE, Voigtländer T, Kreitner KF, Kalden P, Wittlinger T, Scharhag J, Horstick G, Becker D, Hommel G, Thelen M, Meyer J. Quantification of shunt volumes in congenital heart diseases using a breath-hold MR phase contrast technique--comparison with oximetry. Int J Cardiovasc Imaging 2002;18:53-60. [Crossref] [PubMed]
- Colletti PM. Evaluation of intracardiac shunts with cardiac magnetic resonance. Curr Cardiol Rep 2005;7:52-8. [Crossref] [PubMed]
- Rebergen SA, Chin JG, Ottenkamp J, van der Wall EE, de Roos A. Pulmonary regurgitation in the late postoperative follow-up of tetralogy of Fallot. Volumetric quantitation by nuclear magnetic resonance velocity mapping. Circulation 1993;88:2257-66. [Crossref] [PubMed]
- Gelfand EV, Hughes S, Hauser TH, Yeon SB, Goepfert L, Kissinger KV, Rofsky NM, Manning WJ. Severity of mitral and aortic regurgitation as assessed by cardiovascular magnetic resonance: optimizing correlation with Doppler echocardiography. J Cardiovasc Magn Reson 2006;8:503-7. [Crossref] [PubMed]
- Lotz J, Meier C, Leppert A, Galanski M. Cardiovascular flow measurement with phase-contrast MR imaging: basic facts and implementation. Radiographics 2002;22:651-71. [Crossref] [PubMed]
- Garcia J, Kadem L, Larose E, Clavel MA, Pibarot P. Comparison between cardiovascular magnetic resonance and transthoracic Doppler echocardiography for the estimation of effective orifice area in aortic stenosis. J Cardiovasc Magn Reson 2011;13:25. [Crossref] [PubMed]
- Garcia J, Capoulade R, Le Ven F, Gaillard E, Kadem L, Pibarot P, Larose É. Discrepancies between cardiovascular magnetic resonance and Doppler echocardiography in the measurement of transvalvular gradient in aortic stenosis: the effect of flow vorticity. J Cardiovasc Magn Reson 2013;15:84. [Crossref] [PubMed]
- Caruthers SD, Lin SJ, Brown P, Watkins MP, Williams TA, Lehr KA, Wickline SA. Practical value of cardiac magnetic resonance imaging for clinical quantification of aortic valve stenosis: comparison with echocardiography. Circulation 2003;108:2236-43. [Crossref] [PubMed]
- Glockner JF, Johnston DL, McGee KP. Evaluation of cardiac valvular disease with MR imaging: qualitative and quantitative techniques. Radiographics 2003;23:e9. [Crossref] [PubMed]
- Myerson SG. Heart valve disease: investigation by cardiovascular magnetic resonance. J Cardiovasc Magn Reson 2012;14:7. [Crossref] [PubMed]
- Garcia J, Marrufo OR, Rodriguez AO, Larose E, Pibarot P, Kadem L. Cardiovascular magnetic resonance evaluation of aortic stenosis severity using single plane measurement of effective orifice area. J Cardiovasc Magn Reson 2012;14:23. [Crossref] [PubMed]
- O'Brien KR, Gabriel RS, Greiser A, Cowan BR, Young AA, Kerr AJ. Aortic valve stenotic area calculation from phase contrast cardiovascular magnetic resonance: the importance of short echo time. J Cardiovasc Magn Reson 2009;11:49. [Crossref] [PubMed]
- O'Brien KR, Cowan BR, Jain M, Stewart RA, Kerr AJ, Young AA. MRI phase contrast velocity and flow errors in turbulent stenotic jets. J Magn Reson Imaging 2008;28:210-8. [Crossref] [PubMed]
- Defrance C, Bollache E, Kachenoura N, Perdrix L, Hrynchyshyn N, Bruguière E, Redheuil A, Diebold B, Mousseaux E. Evaluation of aortic valve stenosis using cardiovascular magnetic resonance: comparison of an original semiautomated analysis of phase-contrast cardiovascular magnetic resonance with Doppler echocardiography. Circ Cardiovasc Imaging 2012;5:604-12. [Crossref] [PubMed]
- Defining Research – Health Protection Agency. NRES guidance to help you decide if your project requires review by a Research Ethics Committee. Available online: http://www.hpa.org.uk/webc/HPAwebFile/HPAweb_C/1272032326180
- Cawley PJ, Maki JH, Otto CM. Cardiovascular magnetic resonance imaging for valvular heart disease: technique and validation. Circulation 2009;119:468-78. [Crossref] [PubMed]
- Currie PJ, Seward JB, Reeder GS, Vlietstra RE, Bresnahan DR, Bresnahan JF, Smith HC, Hagler DJ, Tajik AJ. Continuous-wave Doppler echocardiographic assessment of severity of calcific aortic stenosis: a simultaneous Doppler-catheter correlative study in 100 adult patients. Circulation 1985;71:1162-9. [Crossref] [PubMed]
- Lee VS, Spritzer CE, Carroll BA, Pool LG, Bernstein MA, Heinle SK, MacFall JR. Flow quantification using fast cine phase-contrast MR imaging, conventional cine phase-contrast MR imaging, and Doppler sonography: in vitro and in vivo validation. AJR Am J Roentgenol 1997;169:1125-31. [Crossref] [PubMed]
- Rodés-Cabau J, Dumont E, Boone RH, Larose E, Bagur R, Gurvitch R, Bédard F, Doyle D, De Larochellière R, Jayasuria C, Villeneuve J, Marrero A, Côté M, Pibarot P, Webb JG. Cerebral embolism following transcatheter aortic valve implantation: comparison of transfemoral and transapical approaches. J Am Coll Cardiol 2011;57:18-28. [Crossref] [PubMed]